Abstract
Cerebrospinal fluid is a fluid covering the brain and spinal cord. Initially thought to be present inside the skull with the purpose to reduce the buoyancy of the brain as well as for its protection, research has shown that it subserves many other important functions such as maintaining intracranial homeostasis, washout of neurotransmitters, providing nutrients to cells. Brain is one of the organs of the body receiving highest cardiac output despite having only 2% of the body weight. The need for high blood flow is due to highest metabolic demand of glucose and oxygen for normal functioning as well as lack of storage capacity. Hence it is understood that the blood flow needs to be maintained within normal limits for optimal functioning, a phenomenon known as cerebral autoregulation. Various aspects of cerebral physiology are discussed.
Keywords
Brain metabolism, Cerebral blood flow, Cerebrospinal fluid, Intracranial pressure, Neurophysiology, Spinal cord
Outline
Intracranial Pressure 62
Introduction 62
Normal Intracranial Pressure 62
Cerebral Compliance 62
Importance of Intracranial Pressure 63
Intracranial Waves 64
Intracranial Wave Analysis 64
Factors Influencing the Intracranial Pressure Waveform 64
Pathological Intracranial Pressure Waves 66
Intracranial Pressure–Derived Indices 66
Intracranial Pressure Monitoring Methods 68
Summary 68
Cerebral Blood Flow 68
Introduction 68
Vascular Anatomy 68
Arterial System 68
Venous System 69
Control of Venous Circulation 70
Regulation of Cerebral Blood Flow 70
Cerebral Autoregulation 70
Mechanisms of Autoregulation 71
Factors Affecting Cerebral Blood Flow 72
Partial Pressure of Arterial CO 2 72
Effects of Partial Pressure of Arterial Oxygen 72
Effects of Hematocrit 73
Effects of Age and Gender 73
Effects of Temperature and Anesthetic Agents 73
Measurement of Cerebral Blood Flow 73
Summary 74
Brain Metabolism 74
Introduction 74
Normal Cerebral Metabolism 74
Brain Energy is Utilized for the Following Process 74
Brain Metabolism in Presence of Oxygen 75
Cerebral Metabolism in Hypoxic State 75
Cerebral Metabolism in Hypoglycaemic States 75
Control of Cerebral Metabolism 76
Metabolic Coupling 76
Metabolic Uncoupling 77
Cerebral Metabolism in Pathological States 77
Apoptosis 78
Monitoring of Cerebral Metabolism 78
Cerebral Microdialysis 78
Summary 79
Cerebrospinal Fluid 79
Introduction 79
Ventricular System 79
Formation of Cerebrospinal Fluid 79
Cerebrospinal Fluid Circulation 80
Cerebrospinal Fluid Absorption 81
Cerebrospinal Fluid Volume and Composition 81
Regulation of Cerebrospinal Fluid Dynamics 82
Effects of Anesthetic Agents 82
Effects of Pathologies on Cerebrospinal Fluid Dynamics 82
Imaging of Cerebrospinal Fluid Pathways 83
Summary 83
The Spinal Cord 83
Introduction 83
Anatomy 84
Organization of the Spinal Cord 84
White Matter of Spinal Cord 84
Spinal Cord Interneurons 86
Glial Cells of Spinal Cord 86
Blood Supply 86
Intrinsic Supply 87
Extrinsic Blood Supply 87
Venous System of Spinal Cord 88
Pathophysiology 88
Autoregulation and CO 2 Reactivity of the Spinal Cord 88
Functions of the Spinal Cord 88
Summary 89
References 89
Intracranial Pressure
Introduction
Brain is enclosed in the skull which is ought to be a protective layer. However, the rigid skull can be damaging the brain in case of pathological lesion. The relationship between the skull contents was initially proposed by Alexander Munro in 1783 and his colleague George Kellie. A modified Munro–Kellie doctrine was proposed by George Burrows in 1846 by including the cerebrospinal fluid (CSF) component along with brain and blood. The current doctrine was introduced by Harvey Cushing who states that in an intact skull , the volume of brain , blood and CSF remains constant . Any increase in the volume of one compartment needs to be compensated by the reduction in either one or both of the other compartments. In normal circumstances the intracranial pressure (ICP) is kept in its normal range, maintaining the relationship between the CSF, blood, and brain tissue constant. Any increase in the ICP can lead to reduced cerebral perfusion and can be harmful. This chapter describes the normal ICP, factors affecting the ICP, and overview of the monitoring of ICP.
Normal Intracranial Pressure
The ICP is derived from the circulating intracranial blood and CSF (ICP total = ICP vascular + ICP CSF ). The vascular component is difficult to quantify due to variation in cerebral blood volume, the autoregulation. The circulatory CSF component may be expressed using Davson’s equation; ICP = (resistance to CSF outflow) × (CSF formation) + (pressure in sagittal sinus). The normal values of ICP in different age groups in horizontal position are 10–15 mmHg for adults and 3–7 mmHg for children. The ICP is lower in newborn (1.5–6 mmHg) due to the opened cranial sutures and fontanels. In the vertical position it is negative with a mean of around −10 mmHg, but not exceeding −15 mmHg. ICP is considered to be high if the value exceeds 20 mmHg and needs to be treated. When the ICP exceeds 60 mmHg, cerebral perfusion ceases and there will be irreversible brain damage and death.
Cerebral Compliance
Since the intracranial volume is inversely proportional to the pressure, a curve can be plotted for change in pressure for a change in volume. This measures the intracranial compliance ( Fig. 4.1 ). The first part of the curve is characterized by a very limited increase in pressure with increase in volume because compensatory reserve is large enough to accommodate the extra volume. With further increasing volume, the compensatory reserve is eventually exceeded, causing a rapid increase in pressure. The normal intracranial compliance is 60–80 mL in young adults and 100–140 mL in older people due to cerebral atrophy. Depending on the compliance the increase in ICP produces various clinical symptoms. Theoder Koher described four stages of raised ICP ( Table 4.1 ).

Stage | Changes in ICP | Clinical Symptoms |
---|---|---|
1 | Increase in tumor volume, compensatory reduction in cerebrospinal fluid and blood volume, no raise in ICP | Usually asymptomatic |
2 | Compensatory mechanisms exhausted, gradual rise in ICP with increase in volume | Drowsiness, headache |
3 | Rapid rise in ICP, falling cerebral perfusion pressure | Deterioration of consciousness, raised blood pressure, bradycardia |
4 | Cerebral vasomotor paralysis, ICP equals mean arterial blood pressure, cerebral perfusion ceases | Coma, dilated fixed pupils, and death |
Importance of Intracranial Pressure
ICP can be raised due to various pathological processes such as brain tumor, trauma, hemorrhage, ischemia producing a stroke or cerebral edema. The raised ICP has two important consequences:
- 1.
The cerebral perfusion pressure (CPP) is denoted by CPP = MAP−ICP, where MAP is the mean arterial pressure and ICP is the intracranial pressure. When the ICP increases, the CPP decreases. In addition, there can be vasomotor paralysis caused by the raised ICP and the intracranial pathological process. Both these factors result in the reduction of CPP causing ischemia and damage to the neurons.
- 2.
The skull is divided into various partial compartments by the folds of dura mater (right and left hemispheres, supratentorial and infratentorial compartments). This leads to compartmentalization of the pressure in case of any pathology. The increase in pressure in one of the compartments pushes the surrounding brain to the other compartments below the dural folds causing herniation of brain. The various herniation syndromes are given in Table 4.2 ( Fig. 4.2 ).
Table 4.2
Various Features of Brain Herniation Syndromes
Herniation
Structures Involved
Clinical Feature
Subfalcine herniation
Cingulate gyrus is pushed below the falx
Dilation of contralateral ventricle
With collapse of ipsilateral ventricle
Uncal herniation
Medial temporal lobe structures are displaced against tentorial edge
Unilateral pupillary dilation with contralateral hemiplegia
Central herniation
Downward displacement of brain stem and diencephalon
Cheyne Stokes respiration, bilateral pinpoint pupils, rapidly progressing to death
Tonsillar herniation
Cerebellar tonsils are pushed downward through foramen magnum
Hydrocephalus, coma, hemodynamic disturbances, and respiratory arrest
Transcalvarial
Brain is pushed against a defect in the skull
Effects depend on the brain area involved
Figure 4.2
Diagrammatic representation of various brain herniation syndromes.
Intracranial Waves
Monitoring of ICP is important as a guide to diagnosis as well as therapeutic interventions. No universally accepted guidelines exist for ICP monitoring, and the indications vary considerably between hospitals and physicians. Some of the indications of ICP monitoring include severe head injury, intracerebral hemorrhage, acute stroke, metabolic encephalopathy, etc.
Intracranial Wave Analysis
Intracranial waveform analysis provides wealth of information regarding the ICP and compliance. It is important to understand the waveform morphology and its changes in different conditions. The pulsations of the CSF are predominant because of transmission of the arterial pulsations. Jugular venous pulsations and respiration also affect the waveform. However, in pathological raise it is mainly the arterial waveform that influences the ICP waves.
Under normotension, normocarbia, and normal PO 2 , the ICP waveform shows two distinct patterns. A slow, large sinusoidal wave and a rapid, small peak wave ( Fig. 4.3 ). The slow sinusoidal pattern corresponds to the phase of respiration ( Fig. 4.3A and B ). The rapid waves correspond to arterial pulse. The rapid wave has an initial peak, followed by dicrotic peak, and few other peaks after the dicrotic peak. These are referred to P1 (percussion wave corresponds to choroid plexus pulsations), P2 (dicrotic wave), and P3 (tidal wave–venous pulsations) waves. In normal persons, P1 amplitude will be higher than P2; however, in patients with raised ICP, P2 will have more amplitude than P1 ( Fig. 4.4 ).


Factors Influencing the Intracranial Pressure Waveform
Various systemic factors can influence the ICP waveforms. It is important to understand the effects of these factors while interpreting the waveforms.
- 1.
Blood pressure : The effects of the changes in blood pressure (BP) depend on the cerebral autoregulation and intracranial compliance. A transient fall in BP causes reduced cerebral blood flow (CBF), fall in CSF production and ICP. However, if the fall is severe or sustained, there will be cerebral vasodilation, brain ischemia, cerebral edema, and rise in ICP. A transient high BP does not affect the ICP within autoregulation. If the BP increases beyond autoregulation, then the CBF will be pressure passive and the ICP will increase ( Fig. 4.3A ). The morphology of the ICP wave changes and the amplitude increases with the shape like arterial pulse waveform.
- 2.
Central venous pressure (CVP) : Elevated CVP causes increase in ICP ( Fig. 4.3B ). The morphology shows more prominent “a” wave peaks.
- 3.
Respiration : During inspiration there is fall in intrathoracic pressure, CVP and is reflected by fall in ICP and vice versa in expiration. In addition, the ICP waveform shows sinusoidal pattern corresponding to respiration ( Fig. 4.3B ).
- 4.
ICP : In case of increase in ICP two distinct changes occur in ICP waveform. The waveform assumes the shape of arterial waves and the venous pulsation disappear. The amplitude of the ICP wave increases. In addition, there will be increase in P2 amplitude compared to P1 wave, and later there will be rounding off without distinct waves. This is called blunting of waves. P1/P2 ratio of more than 0.8 along with an ICP value of more than 10 has been associated with poor compliance of the intracranial compartment. However, there are differences of opinion regarding the predictive value of P1/P2 ratio regarding reduced compliance.
- 5.
Partial pressure of O 2 (PaO 2 ) : In the presence of hypoxia, there was cerebral hyperemia and moderate increase in ICP. The amplitude of the CSF wave increases and the venous pulsations disappear.
- 6.
Partial pressure of CO 2 (PaCO 2 ) : The effects of hypercarbia on the ICP waveform is similar to the pattern seen in increased BP. There will be increased CBF, ICP and the amplitude of the wave increases.
Pathological Intracranial Pressure Waves
The ICP waveform shows three different patterns during increase in ICP. They are of clinical significance.
- 1.
A waves : These are also called plateau waves. A wave comprises a steep rise in ICP from near normal values to 50 mmHg or more, persisting for 5–20 min and then falling sharply. These waves are always pathological and indicate greatly reduced compliance. They are frequently accompanied by neurological deterioration. As the baseline ICP increases the magnitude of A wave also increases. A wave is seen when there are sudden painful stimuli such as endotracheal suctioning to the patient. A waves are associated with severe fall in CPP and needs to be avoided in the ICU care ( Fig. 4.5 ).
Figure 4.5
The Lundberg waves of intracranial pressure (ICP).
- 2.
B waves : These are rhythmic oscillations that occur every 1–2 min. ICP rises in a crescendo manner to levels 20–30 mmHg higher than baseline and then falls abruptly. These waves were originally always associated with Cheyne–Stokes respiration. However, they also occur in ventilated patients and are probably related to changes in cerebrovascular tone and cerebral blood volume. B waves are also indicative of failing intracranial compensation.
- 3.
C waves : These oscillations occur with a frequency of 4–8 per minute and are of smaller amplitude than B waves. They are synchronous with spontaneous Traub–Hering–Meyer type variations in BP and are probably of limited pathological significance.
Intracranial Pressure–Derived Indices
There are two indices which are derived from ICP monitoring: (1) pressure–volume compensatory curve and (2) cerebrovascular pressure reactivity.
Pressure–Volume Compensatory Curve
The pressure–volume compensatory curve measures the degree of compensation that can be achieved due to change in intracranial volume. This measures the relationship between the amplitude (A) of the ICP wave and absolute ICP (P). When the ICP increases the amplitude of the wave also increases; however, the degree of increase in amplitude depends on the intracranial compliance ( Fig. 4.6 ). The curve to the left of the line indicates good compliance, and the change of amplitude to pressure will be nonlinear whereas to the right on the line there is linear increase in both amplitude and pressure.

The index called RAP [correlation coefficient (R) between AMP amplitude (A) and mean pressure (P); which is the index of compensatory reserve] can be derived by calculating the linear correlation between consecutive, time averaged data points of AMP and ICP (usually 40 of such samples are used) acquired over a reasonably long period to average over respiratory and pulse waves (usually 6–10 s periods). This index indicates the degree of correlation between AMP and mean ICP over short periods of time usually 4 min.
Interpretation: If RAP = 0, there is a good intracranial reserve; if RAP = 1, then the compliance is reduced; if RAP <0, the compliance is in steeper part of the curve and autoregulation is lost, the amplitude decreases.
Cerebrovascular Pressure Reactivity
The cerebrovascular reactivity is measured using pressure reactivity index (PRx). It measures the relationship between the slow changes in the arterial BP and ICP. It indirectly measures the autoregulatory state. When the cerebrovascular reactivity is intact, changes in BP cause inverse change in cerebral blood volume and ICP. When the autoregulation is lost, then the increase in BP increases the CBF with a corresponding increase in ICP. PRx is determined by calculating the correlation coefficient between 40 consecutive, time averaged data points of ICP and arterial blood pressure ( Fig. 4.7 ).
Interpretation: When PRx is negative (−1), it indicates good cerebrovascular reserve (Stage 1).
When PRx = 0, the patient is in compensatory part of the ICP curve (Stages 2 and 3).
When PRx is positive (+1), it implies poor reserve (Stage 4).

Intracranial Pressure Monitoring Methods
ICP can be monitored invasively or noninvasively. Invasive methods are more common in use due to their reliability and continuous monitoring. Intraventricular catheter is the gold standard method, whereas fiber optic catheters are more advanced techniques of monitoring. The details of different methods of ICP monitoring will be beyond the scope of this chapter.
Summary
ICP is a key component of the measurement of the intracranial volume/compliance. Many pathological conditions present with features of raised ICP. The symptoms and signs depend on the level of compensation and the duration of the change. Long-standing and slow-growing lesions may produce mild symptoms, whereas severe and acute lesions will rapidly progress to various herniation syndromes and death. Hence it is important to understand the pathophysiology of the disease process and its effect on the ICP so that methods to monitor and treatment plan can be made accordingly.
Cerebral Blood Flow
Introduction
The brain is 2% of body weight. However, it receives 15–20% of cardiac output, utilizes 20% of the oxygen, and 25% of the glucose used by the whole body at rest. The high blood flow is due to high metabolic demand and lack of substrate storage in brain. This high metabolic function is devoted to synaptic activity (50%), maintenance of ionic gradient (25%), and biosynthesis of cellular material and proteins (25%). Hence precise control of CBF is essential for survival. Hence understanding of various factors affecting the CBF becomes important to prevent its fall. Alterations in CBF can be either etiology or contributing factor in many brain pathological conditions. In addition, various drugs and anesthetic agents alter the CBF differently. This chapter reviews the normal blood supply of the brain, factors governing the blood supply, and the effect of various pathological process and drugs.
Vascular Anatomy
Arterial System
Brain is supplied by two internal carotid arteries (anterior circulation) and two vertebral arteries that join to form basilar artery (posterior circulation). The arteries form anastomoses called circle of Willis (CW). The CW is the pyramidal shaped anastomoses in the interpeduncular cistern formed between anterior and posterior circulation as well as between arteries of two cerebral hemispheres ( Fig. 4.8 ). The anastomosis was thought to prevent ischemia if blood supply of any of the major vessels is compromised. However, a recent view on the existence of the CW is it reduces the pressure within the arterial system. Pressure gradient exists because pulse wave and blood flow arrive into the skull through different cerebral arteries asynchronously, due to arterial tree asymmetry. Therefore CW and its communicating arteries protect cerebral artery and blood–brain barrier from hemodynamic stress. The CW is formed by anterior cerebral arteries on either side, anterior communicating artery, internal carotid artery bifurcation, posterior communicating artery, posterior cerebral artery (PCA) on either side. The PCA is a part of posterior circulation. Rest of the arteries mentioned above belongs to the anterior circulation.

Venous System
The cranial veins are classified into three different systems:
- 1.
Superficial—draining the scalp, muscles, and tendons.
- 2.
Intermediate—draining the bone, diploe, and dura mater and consists of emissary veins, diploid veins, meningeal veins, and venous sinuses.
- 3.
Deep system—veins draining the brain.
Normally the flow scalp veins drain mostly to extracranial venous system and partly through emissary veins to dural venous sinus. In case of raised intracranial pressure, there will be retrograde flow from intracranial to extracranial venous system resulting in dilation of scalp veins and emissary veins. The diploid and scalp veins serve as collateral between superior sagittal sinuses to extracranial veins.
The veins of the brain form the superficial and deep venous system. The superficial veins include cortical vein, and they drain to the superior sagittal and transverse sinus. The veins of the medial and inferior cortical surface drain to vein of Galen. There are anastomotic channels between superficial and deep venous systems, which include occipital vein, posterior Calloway vein, and basal vein of Rosenthal. The deep venous system consists of internal cerebral vein, thalamo striate veins, and vein of Galen. The deep venous system drains of inferior sagittal sinus to form straight sinus. The veins of the infratentorial compartment form a network which drains to straight sinus or transverse sinus.
Control of Venous Circulation
The transmural pressure across the intracranial veins is very low, and as the ICP increases the pressure in the cortical veins also increases. There is a cuff system at the junction of the venules where it drains to venous sinuses. The venous sinus pressure remains lower as a result of cuffing of the venules. Animal experiments have found that among the myogenic, metabolic, and neurogenic control, the venous flow is influenced by the sympathetic system especially alpha 1 adrenergic mechanism. During resting conditions 70–80% of the cerebral blood volume is located in the venous system, and it is controlled by cervical sympathetic system. When the ICP increases, the activation of sympathetic system results in veno constriction and drives the blood away thereby offsetting the effects of raised ICP. There is little evidence of metabolic and myogenic control of the venous system. In the presence of hypercapnia, the pial venules dilate in response to increased arterial flow produced by arterial dilation. However, as the PaCO 2 raises further, the increased sympathetic activity causes pial–veno constriction and tries to offset the increased ICP. Acute arterial hypertension causes areas of cortical venous dilation due to increase in the blood flow. The venous transmural pressure may be higher than ICP and can lead to perivenular disruption of blood–brain barrier.
Regulation of Cerebral Blood Flow
The normal CBF is 50–55 mL/100 g/min. The distribution of CBF is not uniform. The gray matter receives approximately 80 mL/100 g/min, whereas white matter receives 20 mL/100 g/min (4:1). Blood flow of less than 20 mL/100 g/min is considered to be critical below which irreversible cellular death can occur. The CBF is affected by many factors ( Fig. 4.9 ). Among them partial pressure of arterial carbon dioxide (PCO 2 ), the MAP, cerebral metabolism, and the autonomic nervous system are the principal regulators of CBF. They do not act independently. There is usually an integration of various factors, which precisely determine the CBF.


Cerebral Autoregulation
Experiments have proved the CBF remains constant despite variation in many local and systemic factors that affect the blood flow. This ability to maintain a constant CBF with wide variation in CPP is called as cerebral autoregulation ( Fig. 4.10 ). Beyond the limits of the autoregulation, the CBF depends on the MAP. However, the limits of autoregulation have been questioned. This questioning is because of the fact that the autoregulation measurement assumes a linear relationship between the CBF and the BP. However, other factors such as baroreceptor reflex, PaCO 2 levels can also affect the validity of the data. In an analysis of transcranial doppler (TCD), MRI, positron emission tomography (PET), etc. based on 41 studies, the authors have found that the cerebrovasculature does have autoregulatory capacity, but its efficacy is not perfect and is dependent on the severity and direction of change in perfusion pressure. Recent data have found that cerebral autoregulation derived from pressure–flow recordings needs to consider not only absolute BP as an input to the cerebral circulation, but also whether BP is accelerating or decelerating and rising or falling. It was found that the compliance of the cerebral vessels also is a major factor in the autoregulation. The brain vessels respond well to rapidly rising BP than a rapidly falling BP.
There are two types of autoregulation described: static and dynamic. “Static” autoregulation refers to a steady-state relationship between MAP and CBF. It is usually assessed using TCD either by changes in the CBF or by changes in MAP by administering phenylephrine. “Dynamic” refers to the cerebral pressure–flow relationship during transient changes in MAP. It is usually measured by transient hyperemic response test. However, many studies have found that the difference between the two is little. Recent methods for assessing the continuous autoregulation include transfer function analysis based on the principle that higher frequency BP fluctuations are more linearly transferred to the cerebral circulation than lower frequency fluctuations.
Mechanisms of Autoregulation
The principle mechanism by which the cerebral vessels respond to change in the MAP is by changing the cerebral vascular resistance (CVR). The initial thought was that the pial arterioles were primarily responsible for CVR; however, it was found that the large intracranial arteries are responsible for changing CVR in response to changes in MAP. The postulated mechanism of autoregulation includes neurogenic, myogenic, and local factors.
Myogenic Control
The myogenic theory described by Bayliss in 1902 proposes that autoregulation is caused by the stretching of the smooth muscle cells of the vessel wall leading to vasoconstriction. The mechanism behind it is by the calcium-mediated pathway in the vascular muscle since it is inhibited by the Ca +2 channel inhibitors. There is an opening of voltage-gated Ca +2 channel following the stretch leading to entry of calcium and vasoconstriction. A calcium-independent mediated theory is also proposed for myogenic action called calcium sensitization, which occurs in the absence of calcium entry.
Neurogenic Control
A large body of data has been published on the role of autonomic nervous system in the control of CBF. The cerebral blood vessels, perivasculature innervations, and astrocyte foot process have been identified as a single unit called “neurovascular unit.” The neurogenic theory suggests that two distinct mechanisms exist in the neural control of CBF. The extrinsic mechanisms are mediated by the superior cervical ganglion (sympathetic), sphenopalatine ganglion (vagus–parasympathetic), and trigeminal ganglion that innervate the cerebral blood vessels from neck to Virchow Robin spaces. The sympathetic stimulation leads to the cerebral vasoconstriction up to the upper limit of CA. The parasympathetic activity has not been fully elucidated. In addition, stimulation of trigeminal nerve leads calcitonin gene-related peptide (CGRP)–mediated vasodilation.
The intrinsic mechanism is caused after the vessels leave the Virchow Robin spaces. The nerves originate from the distal regions of the brain as well as local interneurons which connect to the astrocyte foot process rather than the blood vessels. These nerves control constriction or dilation of the vessels depending on the stimuli. The exact mechanism is not understood. GABA-mediated vasodilation occurs in the interneurons.
Flow Metabolism Coupling
Even though the brain is only 2% of body weight, it consumes 20% of energy of the whole body. Another unique feature of the brain energy consumption is the regional differences in the synaptic activity. Much of the energy expenditure is used maintaining the ion homeostasis. Sherrington in the 19th century proposed that the brain possesses an intrinsic ability to maintain its local blood flow depending on its need which is otherwise its activity. This intrinsic ability is called “flow metabolism coupling” ( Fig. 4.9 ).
Brain is unique in that different function is represented in specific areas. Initiation of a particular function such as motor movements, speech, etc. results in increase in the blood flow in that area subserving the function. This is the principle used in functional MRI using blood-oxygen-level dependent contrast imaging signals. The increase in blood flow was thought to be due to initiation of action potentials. However, studies have shown that increased synaptic transmission during activity leads to increase product of metabolism either adenosine or changes in ions like K + and H + ions. Potassium ions released during synaptic transmission have been shown to act via K + -dependent ATP channels in the vascular smooth muscle causing vasodilation. Hydrogen ions also produce vasodilation, but the exact mechanism is not known. It is probably thought to be similar to CO 2 -induced vasodilation or via nitric oxide pathways. Among the metabolites, adenosine appears to be a potent vasodilator. Extracellular levels of adenosine were found to increase with neuronal activity, and topical application of adenosine to cerebral microcirculation causes vasodilation.
Factors Affecting Cerebral Blood Flow
Partial Pressure of Arterial CO 2
Changes in the PaCO 2 affect the CBF as cerebral circulation is very sensitive to the levels of PaCO 2 . Studies have shown an approximate 3–6% increase and/or 1–3% decrease in flow per mmHg in PaCO 2 above and below eucapnoeic PaCO 2 , respectively. All the vessels of cerebral circulation such as large arteries of the neck, intracranial vessels, pial arterioles are sensitive to changes in PaCO 2 . The CO 2 reactivity of the microvasculature in gray matter is greater than that of white matter because of relatively less vascularization of white matter. The mechanism by which PaCO 2 acts is not known. It is thought to exert action by directly acting on the smooth muscle of the blood vessels or by changes in local tissue pH. The actions of PaCO 2 occur regardless of blood pH.
An increase in PaCO 2 causes cerebral vasodilation and hypocapnia causes vasoconstriction. The lower limit of CA has been found to shift upwards in acute hypercapnia. The lower limit of CA of 50–60 mmHg under normocapnia (PaCO 2 = 30–50 mmHg) was shifted to 80–100 mmHg when hypercapnia (PaCO 2 = 70–95 mmHg) was instituted. The upper limit in hypercapnia was found to be limited leading to a situation in which the CA limits were shorter in hypercapnia conditions. In severe hypercapnia, the blood flow is pressure dependent. It is also found that PaCO 2 of more than 55 mmHg abolishes CA ( Fig. 4.11 ).
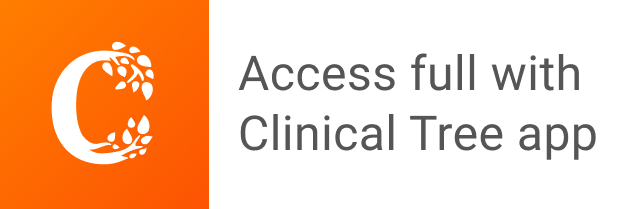