Neuromuscular blocking agents (NMBAs) were first “discovered” by the native Indian populations of South America and were used for hunting game. They called their plant-based concoction “ourari,” which was later interpreted as “curare” by the early European explorers. The use of neuromuscular relaxants in medicine, however, would have to wait until the mid-1800s, when Dr Louis Sayres of New York attempted to treat the spasms associated with tetanus with a rudimentary curare preparation (Chapter 10). The first successful use of curare during surgery was described by Dr Arthur Lawen in 1912; however, it would take an additional 30 years of further refinement in anesthesia methodology, notably improved tracheal intubation techniques, before Drs Harold Griffith and Enid Johnson demonstrated successful and safe use of curare in surgery and anesthesia. After their groundbreaking work, research into NMBAs led to the development and purification of several different neuromuscular agents. Of the modern neuromuscular agents still in clinical use, succinylcholine was first synthesized in 1906, but its clinical effect was not recognized until 1949. Pancuronium was manufactured in 1964, and further research to lessen its side-effect profile led to the development of vecuronium in 1979. More recently, mivacurium and rocuronium became available for clinical use in the early 1990s.
Further research into compounds with more rapid metabolism and elimination resulted in the introduction into practice in the 1980s of vecuronium,1 an aminosteroid, and atracurium,2,3 a benzylisoquinolinium compound. These relaxants had little or no dependence on the kidney for elimination, and vecuronium lacked cardiovascular effects.1 The degradation of atracurium via Hofmann elimination removed any important influence of advanced age or organ failure on the profile of the drug and greatly increased its acceptance in the clinical setting, despite its hemodynamic side-effect profile. In an attempt to decrease the histamine release associated with atracurium, one of its isomers, cisatracurium, was isolated in the mid-1990s and became widely popular in anesthesia practice.
NMBAs act to prevent effective transmission of nerve impulses across the neuromuscular junction, the synapse interposed between the presynaptic nerve terminal and the postsynaptic muscle membrane. Under normal conditions, when a nerve impulse is transmitted along the axon and reaches the nerve terminal, it causes release of stored acetylcholine from the nerve terminal (readily releasable vesicle pool). The acetylcholine (released as quanta, each quantum containing approximately 5000 acetylcholine molecules per vesicle) then diffuses across the synaptic cleft and interacts with nicotinic acetylcholine receptors on the postsynaptic (muscle) membrane. When enough of these receptors are activated (all-or-none rule), an action potential ensues leading to muscle contraction. The adult acetylcholine receptor (Figure 9–1) consists of 2 identical α subunits, and 1 β, δ, and ε subunits each, arranged in a rosette pattern (α2βδε). In fetal acetylcholine receptors, the ε subunit is replaced by a γ subunit (α2βδγ) (Figure 9–2). Acetylcholine must bind to both α subunits simultaneously in order to induce the conformational change needed to activate the receptor. The activated (open) receptor allows for the flow of sodium, calcium, and potassium ions across their electrochemical concentration gradients, resulting in muscle membrane depolarization and muscle fiber contraction.
Figure 9–1
Adult acetylcholine receptor (AChR) consisting of 5 subunits (two identical α subunits, and one β, δ, and ε subunits each) arranged in a rosette (α2βδε) pattern. The two α subunits contain the recognition sites for acetylcholine binding. (Reproduced with permission from Brull SJ, Naguib M. Review of Neuromuscular Junction Anatomy and Function. In:The Neuroscientific Foundations of Anesthesiology. Mashour GA, Lydic R (Editors), Oxford University Press, New York. 2011; pp:205-210.)

Figure 9–2
Fetal acetylcholine receptor (AChR) consisting of 5 subunits (two identical α subunits, and one β, δ, and γ subunits each) arranged in a rosette pattern. Note that the ε subunit is replaced by a γ subunit resulting in (α2βδγ) pattern. The two α subunits contain the recognition sites for acetylcholine binding. (Reproduced with permission from Brull SJ, Naguib M. Review of Neuromuscular Junction Anatomy and Function. In:The Neuroscientific Foundations of Anesthesiology. Mashour GA, Lydic R (Editors), Oxford University Press, New York. 2011; pp:205-210.)

Currently, there are 2 classes of NMBAs available in the clinical setting. Each prevents muscle contraction through different mechanisms.
Succinylcholine chloride (suxamethonium chloride) is the only depolarizing agent available for clinical use today. Succinylcholine acts by binding to one or both of the α subunits of the postsynaptic receptor, leading to activation of the end-plate receptor (see Figure 9–1). Since the elimination of succinylcholine from the neuromuscular junction is slower than that of acetylcholine (a few milliseconds), the effect is that of prolonged depolarization. During this period of depolarization, initial disorganized muscle contraction (evidenced clinically by muscle fasciculations) is followed by flaccid paralysis. While depolarized, the receptor is not susceptible (ie, it is desensitized) to further stimulation by acetylcholine until succinylcholine redistributes away from the neuromuscular junction. Depolarizing neuromuscular blockade is also referred to as phase I neuromuscular block. If large doses of succinylcholine are administered, or if the patient has an abnormal (“atypical”) gene for butyrylcholinesterase, the patient may develop a neuromuscular block with the characteristics of that produced by nondepolarizing NMBAs, called phase II neuromuscular block. Table 9–1 describes the differences between phase I and phase II block. However, it has been shown that post-tetanic potentiation and presence of fade in response to train-of-four and tetanic stimuli (ie, phase II block) may also be characteristics of neuromuscular block after bolus administration of different doses of succinylcholine.4 It appears that some characteristics of phase II blockade are evident following the administration of an initial dose of succinylcholine—as small as 0.3 mg/kg.4
Succinylcholine is a white, odorless, crystalline powder that is readily soluble in water. In powder form, it is stable indefinitely at room temperature. Once mixed in solution, it is relatively unstable in alkaline solutions but becomes more stable in acidic solutions. For this reason, the pH of succinylcholine solution is adjusted to 3.5 to 4 by the addition of hydrochloric acid. The stability is further enhanced by refrigeration, which helps preserve its original potency. Succinylcholine is marketed premixed in solutions of 20 mg/mL for bolus administration; it is also supplied in 50-mg/mL and 100-mg/mL concentrations for preparing an infusion mixture. Additionally, succinylcholine chloride powder (chloride dihydrate) for infusion is available in 500-mg or 1000-mg vials.
Succinylcholine is an ultra–short-acting agent that is useful for inducing skeletal muscle relaxation quickly; however, a number of significant side effects need to be considered prior to its use. Succinylcholine is usually administered intravenously, but intraosseous,5,6 intralingual,7 and intramuscular8 administration has been reported in special circumstances such as in patients with laryngospasm without preexisting intravenous access. The clinical utility of some of the aforementioned approaches has been debated.
Succinylcholine is a long, flexible molecule that is comprised of 2 molecules of acetylcholine joined end-to-end via their terminal acetate methyl groups. It is positively charged and has low lipid solubility, giving it a volume of distribution roughly equivalent to the extracellular space. The potency of NMBAs is quantified by the dose required to decrease the strength of contraction in the adductor pollicis muscle (thumb adduction) by a certain percent from baseline. This is known as the effective dose (ED). Thus, the ED50 or ED95 is the dose of muscle relaxant required to decrease the strength of contraction by 50% or 95% from baseline, respectively. In some studies, the estimated ED95 of succinylcholine was 0.63 mg/kg.9 Using cumulative dose-response techniques, Kopman10 estimated that its potency to be far greater, with an ED95 of less than 0.3 mg/kg. The usual succinylcholine intubating dose in adults is 1 to 1.5 mg/kg intravenously (3 to 5 times the ED95). When administered intramuscularly or to children, higher doses, in the range of 2 to 4 mg/kg, are often required. Administration of a defasciculating dose of a nondepolarizing neuromuscular blocking agent, such as d-tubocurarine, increases the ED95 requirement by roughly 50% and therefore a larger dose of succinylcholine may be needed.9
Figure 9–3 shows a simulation-derived example of the plasma concentration, effect-site concentration, and recovery from intravenous administration of 1.0 mg/kg or 1.5 mg/kg of succinylcholine. Following administration of an intubating dose of succinylcholine, profound paralysis occurs within 1 to 2 minutes, with spontaneous recovery occurring within 10 to 15 minutes.11,12, and 13 The central muscles, such as the laryngeal adductor muscles, are affected faster than the peripheral muscles.14 This may be due to rich blood flow to the central muscles.
Hydrolysis of succinylcholine by butyrylcholinesterase (also known as plasma pseudocholinesterase or plasma cholinesterase) occurs in plasma, follows first-order kinetics, and occurs rapidly in most patients. In fact, the majority of administered succinylcholine (up to 90% of the administered dose) is hydrolyzed by butyrylcholinesterase to succinylmonocholine and choline before reaching the neuromuscular junction.15 Succinylmonocholine is known to possess a weak neuromuscular blocking ability. The elimination half-life (t½β) of intravenous 0.5, 1.0 or 5.0 mg/kg succinylcholine is about 5 minutes in dogs, with a distribution half-life (t½α) of less than 1 minute.16 In humans, the mean pharmacokinetic parameters of 1 mg/kg succinylcholine are apparent volume of distribution = 16.4 mL/kg, total body clearance = 40.5 L/min, and t½β = 16.6 s.17 In contrast to succinylcholine, succinylmonocholine has a delayed onset of peak plasma concentration, slower distribution, and longer t½β of 1 to 3 hours.18
Acetylcholinesterase, which rapidly degrades acetylcholine, does not have the ability to hydrolyze succinylcholine, and the termination of the effect at the neuromuscular junction is largely due to redistribution of succinylcholine away from the neuromuscular junction along its concentration gradient into the plasma. Patients with abnormal butyrylcholinesterase activity can have significantly prolonged duration of action of succinylcholine that is correlated with butyrylcholinesterase activity.19
Normal individuals have 2 functional genes located on chromosome 3 that encode for pseudocholinesterase. It is estimated that 1 in 25 patients may be heterozygous “atypical,” and 1 in 2500 individuals are homozygous “atypical” for the pseudocholinesterase gene.20,21 These latter patients can have varying degrees of sensitivity to succinylcholine and may require prolonged postoperative mechanical ventilation in some cases. Quantification of the degree of abnormality (ie, enzymatic activity) can be obtained using dibucaine number testing. Dibucaine is a local anesthetic that inhibits normal butyrylcholinesterase activity to a greater degree than atypical butyrylcholinesterase. This measurement, referred to as the dibucaine number, is an approximation of the percentage of normal enzyme inhibition. In normal individuals, the dibucaine number is approximately 80, and for homozygous abnormal individuals, the dibucaine number is approximately 20. For heterozygous individuals, the dibucaine number can be anywhere between 20 and 80 but tends to cluster in the range of 40 to 60. Fluoride-resistant butyrylcholinesterase variants have also been described. The fluoride number indicates the percentage inhibition of butyrylcholinesterase in the presence of fluoride.
In addition to inherent enzyme abnormalities, a host of chronic disease states can result in decreased pseudocholinesterase synthesis and function. Liver disease, renal disease, acute burns, and sepsis have all been associated with decreased production of pseudocholinesterase. Pregnancy has also been associated with decreased butyrylcholinesterase activity and decreased absolute quantity (due to salt and water retention).22,23 Although most genetic variants of serum butyrylcholinesterase are associated with decreased activity, some rare variants are associated with increased enzyme activity (2 to 3 times normal).24
Succinylcholine is considered by many to be the drug of choice for muscle relaxation when performing an anesthetic rapid-sequence induction and intubation. In patients who have recently consumed a meal or have certain medical conditions (eg, small bowel obstruction, severe gastroesophageal reflux disease), there is a risk of vomiting and aspiration of gastric contents into the lungs during induction of anesthesia and tracheal intubation. Because of succinylcholine’s fast onset of action and high reliability in producing profound neuromuscular block (thus providing optimal intubating conditions), tracheal intubation with a cuffed tracheal tube can be achieved rapidly, thereby decreasing the likelihood of pulmonary aspiration.
Succinylcholine can also be administered via infusion in cases where profound, short-duration paralysis is needed. During such cases, succinylcholine continuous infusion should be administered via a dedicated intravenous catheter to prevent an accidental bolus and/or the development of phase II block. Continuous neuromuscular monitoring should routinely be employed along with careful titration to prevent complete loss of muscle response to stimulation. This continuous succinylcholine infusion technique, however, has lost its popularity with the introduction of the newer, intermediate-acting neuromuscular blockers.
The therapeutic benefits of succinylcholine should always be weighed against the side effects associated with its use. While still useful clinically for rapid-sequence induction and intubation, research into newer nondepolarizing NMBAs with short duration may eventually contribute to rendering succinylcholine obsolete.
Muscle fasciculations following administration of succinylcholine are very common and were first described more than 60 years ago.25 Fasciculations are due to retrograde propagation of the action potential to the prejunctional terminal of the motor neuron (resulting in disorganized, muscle fiber contractions) and may be prevented by administering a “defasciculating” dose of nondepolarizing NMBA several minutes prior to succinylcholine. Another method of preventing or attenuating fasciculations is the “self-taming” technique, in which 5 to 10 mg of succinylcholine precedes administration of the intubating dose of succinylcholine. Administration of the defasciculating dose, however, is not devoid of potential complications; it has been associated with symptoms of muscle weakness and even respiratory paralysis, so the clinician must be ever vigilant and prepared to secure the airway emergently.
Myalgias have also been associated with succinylcholine administration, and the incidence reported in the literature varies between 2% and 89%.26,27 Females are more likely to experience postsuccinylcholine myalgias than men, while pregnancy and the extremes of age (children and adults older than 60 years of age) appear to be somewhat protective.28,29 Interestingly, the frequency and severity of myalgias appear to be inversely proportional to the state of muscular fitness, such that athletes will experience fewer side effects.30 Similarly, early ambulation postoperatively has been reported to increase the incidence and severity of myalgias.31 While myalgias are thought to occur from microtrauma during fasciculations (perhaps due to the asynchronous contraction of adjacent fascicles without the opportunity for shortening of the fiber length and resulting in fiber rupture that causes pain), they are not directly related to the severity of the fasciculations themselves.32 In many (but not all) cases, a defasciculating dose of nondepolarizing NMBA seems to be successful in ameliorating the pain associated with myalgias. Pretreatment with a prostaglandin inhibitor (lysine acetyl salicylate) has been shown to be effective in decreasing the incidence of muscle pains after succinylcholine.33 This suggests a possible role for prostaglandins and cyclooxygenases (COX) in succinylcholine-induced myalgias. Several other classes of drugs (benzodiazepines, local anesthetics, vitamin C, dantrolene, calcium gluconate, magnesium, anticonvulsants, and nonsteroidal anti-inflammatory drugs) have been administered in an attempt to decrease postoperative myalgias with varying degrees of success.
The use of succinylcholine has been associated with increases in intraocular, intragastric, and intracranial pressures. The increase in intragastric pressure is thought to be due to the contractions of the abdominal wall musculature. Fortunately, this pressure increase is offset by a concomitant contraction of the lower esophageal sphincter, and reflux is prevented. Similarly, intracranial pressure is increased by succinylcholine, although the effect is mild and probably negligible when compared to the stimulating effects of laryngoscopy and intubation on intracranial pressures. The increase in intragastric and intracranial pressures can be attenuated by pretreatment with a nondepolarizing NMBA. Intraocular pressure (IOP) transiently increases by an average of 8 mm Hg following administration of succinylcholine, an increase that was feared detrimental to patients with open-globe injury. The exact etiology of the increase has not been fully elucidated and is not consistently prevented by administration of a defasciculating dose of nondepolarizing NMBA.34 Extrusion of intraocular contents due to succinylcholine-induced increase in IOP, a much-feared complication, has never been reported, however. In fact, other maneuvers increase the IOP to a much greater degree: eye blinking increases IOP by 10 to 50 mm Hg,35 coughing or vomiting increase IOP by 30 to 40 mm Hg, and pressure from the face mask during assisted ventilation may increase IOP by hundreds of mm Hg (Figure 9–4). Several studies of thousands of patients with open-globe injuries have failed to report any extrusion of intraocular contents from succinylcholine use.36
Figure 9–4
Mean increase in intraocular pressure (IOP) from baseline (normal = 10 mm Hg) in response to various procedures and maneuvers. The numbers in parentheses refer to the following studies:
1.
Murphy DF. Anesthesia and intraocular pressure. Anesth Analg. 1985;64:520530.2.
Lamb K, James MF, Janicki PK. The laryngeal mask airway for intraocular surgery: effects on intraocular pressure and stress responses. Br J Anaesth. 1992;69:143147.3.
Drenger B, Pe’er J. Attenuation of ocular and systemic responses to tracheal intubation by intravenous lignocaine. Br J Ophthalmol. 1987;71:546548.4.
Miller D. Pressure of the lid on the eye. Arch Ophthalmol. 1967;78:328330.5.
Rafuse PE, Mills DW, Hooper PL, Chang TS, Wolf R. Effects of Valsalva’s manoeuvre on intraocular pressure. Can J Ophthalmol. 1994;29:7376.6.
Duncalf D, Weitzner SW. The influence of ventilation and hypercapnea on intraocular pressure during anesthesia. Anesth Analg. 1963;42;232237.7.
Kelly RE, Dinner M, Turner LS, Haik B, Abramson DH, Daines P. Succinylcholine increases intraocular pressure in the human eye with the extraocular muscles detached. Anesthesiology. 1993;79:948952.8.
Bithal PK, Reddy TS, Prabhakar H. Effect of repeat laryngoscopy on intraocular pressure. Eur J Anaesthesiol. 2004;21:496497.9.
McGoldrick KE, Gayer SI. Anesthesia for Ophthalmic Surgery (Chapter 51). In: Barash PG, Cullen BF, Stoelting RK, Cahalan MK, Stock MC, eds. Clinical Anesthesia. 6th ed. Philadelphia: Lippincott Williams & Wilkins;, 2009:13211345.10.
Coleman DJ, Trokel S. Direct-recorded intraocular pressure variations in a human subject. Arch Ophthalmol. 1969;82:637640.ETT, endotracheal tube; LMA, laryngeal mask airway.
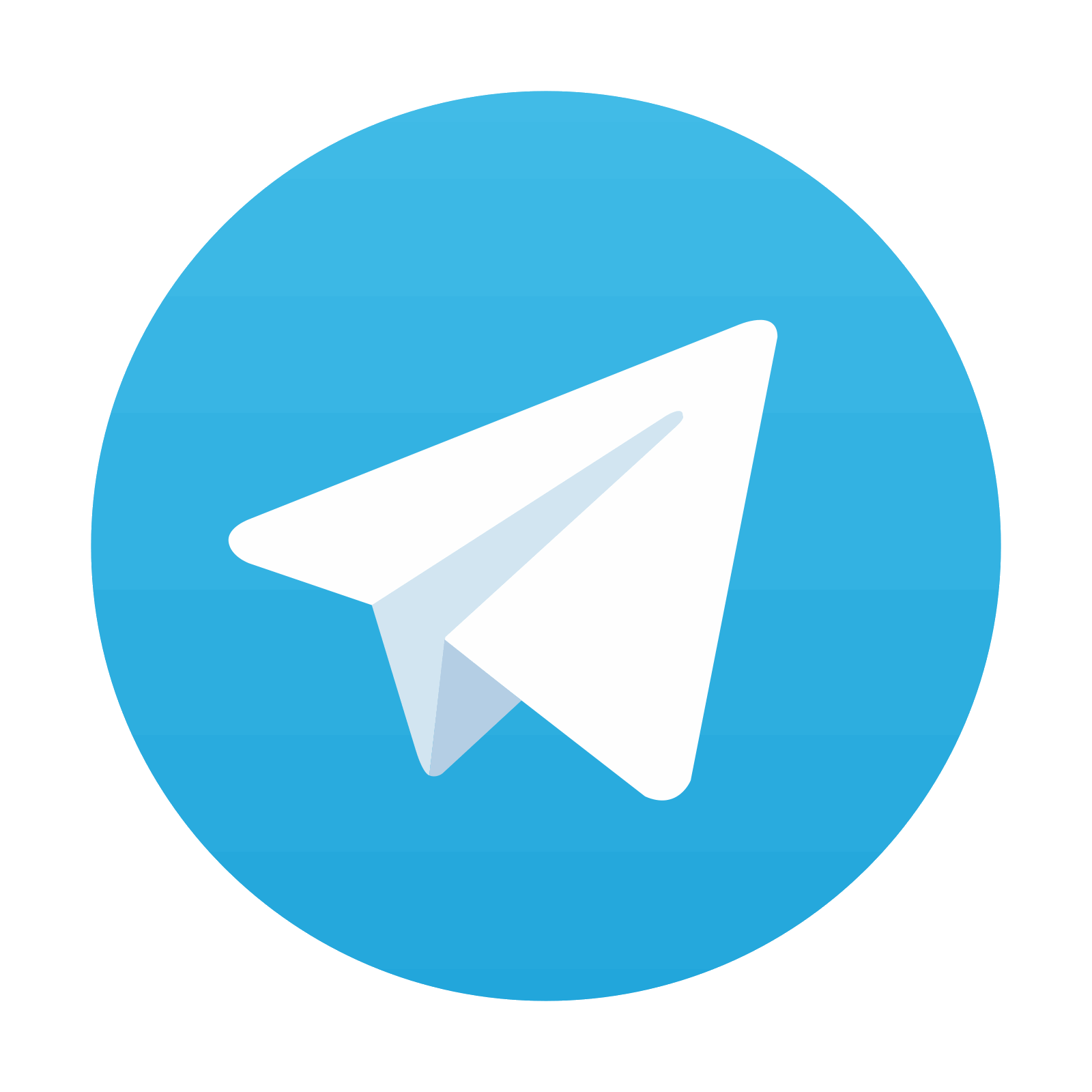
Stay updated, free articles. Join our Telegram channel
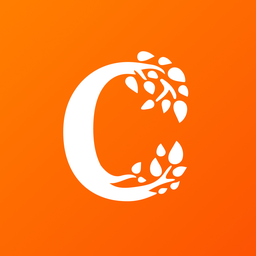
Full access? Get Clinical Tree
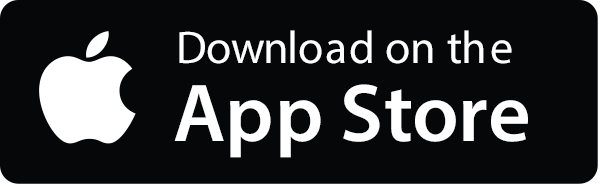
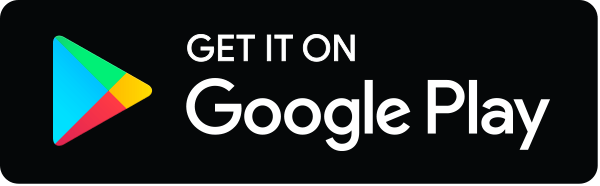
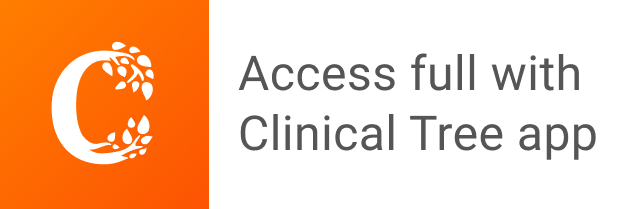