FLOW–METABOLISM COUPLING
In the normal brain, an increase in cerebral metabolism is rapidly matched by local increases in CBF. This is referred to as regional flow–metabolism coupling or cerebral metabolic autoregulation (2). CBF is thus linked to brain function and metabolism so that CBF varies in parallel with CMRO2 (Fig. 28.2). Autoregulation and increased oxygen extraction are two compensatory responses to acute reductions in CBF (3–5). Oxygen extraction is able to vary within a narrow range. Misery perfusion occurs when oxygen extraction is increased as a response to increased CMRO2, either when autoregulatory CBF compensation has been exceeded or uncoupling has occurred (6). As cerebral perfusion pressure (CPP) falls, CBF is maintained initially by resistance arteriole vasodilation (7). Severe ischemia results as CPP is further reduced; the capacity of CBF autoregulation and increased oxygen extraction is exhausted, and CBF falls as a function of pressure. Positron emission tomography (PET) studies indicate that this occurs with relatively preserved CMRO2 in the penumbra of a focal ischemic area.
Several vasoactive metabolic mediators have been proposed for cerebral regulation, including hydrogen ion, potassium, CO2, adenosine, glycolytic intermediates, phospholipid metabolites (2), and, more recently, nitric oxide (8). In humans, flow–metabolism coupling is evident during a variety of motor and cognitive tasks that can be mapped using CBF techniques (9).
The global relationship between CBF and CMRO2 can be expressed by the Fick equation where DajO2 is the arteriojugular difference in oxygen content:
CMRO2 = DajO2 × CBF or DajO2 = CMRO2/CBF
In brain injury, during hypothermia, and under the influence of anesthetic agents, CBF and metabolism may become dissociated. In a series of 109 severe head injury patients, Bouma et al. reported that CBF measured within the first 6 hours after trauma was less than 18 mL/min/100 g (i.e., the threshold for cerebral ischemia) in one-third of the patients (10). Arterial vasospasm was an independent predictor of poor outcome (11). Secondary ischemic neurologic damage associated with systemic factors (e.g., hypotension or hypoxemia) and local factors (e.g., intracranial hypertension, hypoperfusion) worsened outcome. Disruption of normal homeostatic mechanisms such as pressure autoregulation (see below) may also aggravate cerebral ischemia. Mechanical hyperventilation used to reduce intracranial pressure (ICP) may be deleterious by decreasing CBF, and may thus also lead to ischemia (12).
Hypothermia
Cerebral protection by hypothermia is commonly attributed to cerebral metabolic suppression. The temperature coefficient (Q10) is the factor by which CMRO2 is decreased by a 10°C decrease in temperature. Between 37°C and 27°C, the temperature coefficient is 2.2, but between 27°C and 17°C—a temperature range during which electroencephalographic activity ceases—the temperature coefficient doubles to 4.5. Below 17°C, the Q10 returns to near 2.0 (Fig. 28.3). In the absence of electroencephalographic activity (e.g., during barbiturate coma), however, the Q10 remains near 2.0 over the entire temperature range. With moderate hypothermia (i.e., above 27°C), both CO2 reactivity and autoregulation are intact while CBF and CMRO2 remain coupled (13). Evidence suggests that there is a change in the coupling of blood flow and metabolism during deep cerebral hypothermia (below 25°C). Nonetheless, metabolic regulation remains a main determinant of CBF even during deep cerebral hypothermia (14).

Figure 28.1. Magnetic resonance images from a patient with a glioblastoma multiforme. The axial, gadolinium-enhanced T1-weighted image demonstrates the enhancing tumor margin with its nonenhancing central necrosis. The axial, T2-weighted image shows water as a bright signal in perifocal edema and the central tumor necrosis, as well as in the cerebrospinal fluid, and the fluid attenuation inversion recovery image highlights only the edema around the tumor and suppresses the cerebrospinal fluid signal and the tumor necrosis. (Courtesy of Ilona Schmalfuss, MD.)

FIGURE 28.2. Flow–metabolism coupling in the central nervous system. As the metabolic needs of the brain, expressed as the cerebral metabolic requirement for oxygen (CMRO2), increases, cerebral blood flow increases in parallel.
Anesthetics
With the exception of ketamine, most intravenous and inhalational anesthetics depress cerebral metabolism (15,16), with consequent reductions in oxygen consumption (CMRO2), CBF, and ICP with intact autoregulation (17). As CMRO2 decreases, CBF is reduced proportionately because of flow–metabolism coupling. Flow–metabolism coupling usually remains intact after the administration of sodium thiopental and propofol (17), and cerebral oxygen saturation is expected to remain unaltered or improve. Etomidate, in contrast, can produce a rapid reduction in CBF accompanied by a slower reduction in CMRO2 (18,19). This flow–metabolism coupled mismatch, resulting from a greater reduction in flow than demand, may induce significant, albeit transient, cerebral oxygen desaturation.
Propofol is believed to maintain cerebral autoregulation, and even high doses of this drug do not obtund autoregulation or carbon dioxide reactivity (20). The effect of propofol on flow–metabolism coupling is more controversial, with at least one study demonstrating intact coupling (21). Both increased and decreased cerebral oxygen extraction have been demonstrated with propofol, suggesting CBF–CMRO2 uncoupling (22,23). Despite the fact that the retention of normal flow–metabolism coupling is thought to occur in only a proportion of head-injured patients, there is a paucity of data regarding the influence of propofol on flow–metabolism coupling after traumatic brain injury (TBI). It has been demonstrated that after TBI, flow–metabolism coupling remains intact during a step increase in propofol infusion rates (24), as is the case in noninjured patients (25).
Benzodiazepines and opiates appear to have limited intrinsic effects on CBF, CMRO2, and CBF–CMRO2 coupling (26,27). Because of their sedative properties, they cause a decrease in CBF and ICP that parallels the sedation-induced decrease in CMRO2. As with all anesthetics, the decreased sympathetic tone caused by the sedation, on the other hand, risks a decrease in mean arterial pressure that may in fact diminish cerebral perfusion. Dexmedetomidine, an α2-receptor agonist, is a recent and relatively expensive sedative. Similar to opiates and benzodiazepines, its effects on cerebral physiology appear to be caused by the sedation (28). Limited experience in traumatic brain-injured patients did not reveal any adverse effects (29).
Arterial Carbon Dioxide and Oxygen
Carbon dioxide is a potent cerebral vasodilator and thus a major determinant of CBF (Fig. 28.4) (30). At normotension, CBF increases almost linearly when the arterial partial pressure of carbon dioxide (PaCO2) increases from 25 to 80 mmHg. Global CBF varies 2% to 4% for each millimeter of mercury change in PaCO2 (31). The effects of PaCO2 on cerebral circulation are regulated by a complex and interrelated system of mediators. The initial stimulus of CO2-induced vasodilation is a decrease in brain extracellular pH (32), further mediated by nitric oxide, prostanoids, cyclic nucleotides, potassium channels, and a decrease in intracellular calcium concentration as a final common pathway.
Arteriolar tone has an important influence on how PaCO2 affects CBF. Moderate hypotension impairs the response of the cerebral circulation to changes in PaCO2, while severe hypotension abolishes it altogether (33). Similarly, PaCO2 modifies pressure autoregulation, and from hypercapnia to hypocapnia, there is a widening of the autoregulation plateau (34). The response of cerebral vessels to CO2 can be used therapeutically by instituting hyperventilation to decrease CBF, in turn reducing cerebral blood volume and ICP. Numerous studies on CO2 reactivity have generally demonstrated that the response is preserved during intravenous or inhalation anesthesia (35). CO2 reactivity has also been used to assess the adequacy of brain perfusion in patients with internal carotid artery stenosis or cerebrovascular disease. In severe head injury, intact CO2 vasoreactivity is a good predictor of the effectiveness of hyperventilation or barbiturate therapy in controlling elevated ICP in individual patients (36). Furthermore, impaired cerebral CO2 vasoreactivity is associated with a poor outcome in patients with severe head injury (37). On the other hand, hyperventilation has been found to increase oxygen extraction, cause misery perfusion, and thereby promote secondary brain injury (12).

Figure 28.3. Theoretical interaction of temperature, brain function, metabolic requirements (CMRO2), and calculated Q10 values. During temperature reduction from 37° to 27°C, function is maintained, and metabolism devoted to function and maintenance of integrity are presumed to be equally affected, with a slightly more than 50% reduction in CMRO2 generating a Q10 value of 2.4. A further 10°C reduction in temperature to 17°C abolishes function, resulting in a step decrease in CMRO2 such that the calculated Q10 value is 5.8. At this point, the total oxygen consumed by the brain is reduced to less than 8% of the normothermic value. (With permission from Black S, Michenfelder JD. Cerebral blood flow and metabolism. In: Cucchiara RF, Black S, Michenfelder JD, eds. Clinical Neuroanesthesia. 2nd ed. New York: Churchill Livingstone; 1998.)

FIGURE 28.4. Effect of arterial CO2 on cerebral blood flow.
Moderate changes in arterial PO2 (PaO2) do not significantly alter CBF. When PaO2 falls below 50 mmHg, however, CBF increases so that cerebral oxygen delivery remains constant (30). Hypoxia acts directly on cerebral tissue to release lactic acid, adenosine, and prostaglandins, which contribute significantly to cerebral vasodilation. Hypoxia also acts directly on cerebrovascular smooth muscle to produce hyperpolarization and reduce calcium uptake, both mechanisms enhancing vasodilation.
Pressure Autoregulation
Pressure autoregulation refers to the ability of the brain to maintain total and regional CBF nearly constant despite large changes in systemic arterial blood pressure (Fig. 28.5), independent of flow–metabolism coupling (34). Autoregulation is generally expressed as the relationship between CBF and arterial blood pressure when cerebral venous and CSF pressures are low. It can be more precisely defined using the relationship between CBF and CPP that represents the difference between mean systemic arterial pressure and cerebral outflow pressure. Because the cerebral venous system is compressible and may act as a “Starling resistor” or waterfall phenomenon (38), outflow resistance is governed by whichever pressure is higher—CSF pressure (ICP) or venous outflow pressure (jugular bulb pressure).

FIGURE 28.5. Preserved cerebral pressure autoregulation (solid line) keeps cerebral blood flow constant over a wide range of perfusion pressures. Impaired autoregulation (dashed lines) manifests as a shortened or even absent plateau of the autoregulation curve.
The cerebral vascular resistance (R) can be expressed as:
R = CPP/CBF = (8/π) × h × (l/r4)
where (8/π) is a constant for calculation, h = blood viscosity, l = length, and r = radius of the vessel. Importantly, the radius enters to the fourth power in the equation, making it the most efficient means of controlling vascular resistance.
In adults under normal conditions, CBF remains constant between a CPP of roughly 60 and 150 mmHg (34). The autoregulation curve is shifted to the right in hypertensive patients and to the left in neonates. At the lower limit of autoregulation, cerebral vasodilation is maximal, and below this level, CBF falls passively with CPP. Beyond the upper limit where vasoconstriction is maximal, the elevated intraluminal pressure may force the vessels to dilate, leading to an increase in CBF and damage to the blood–brain barrier (34,39). Metabolic mediators, such as adenosine, can also be involved in the low-pressure range of autoregulation (39).
Pressure autoregulation can be impaired in many pathologic conditions, including brain tumor, subarachnoid hemorrhage, stroke, or head injury. A loss of CBF regulatory capacity can be attributed to damage of the control system (e.g., cerebral vessels)—usually referred to as “paralysis” in the clinical literature (40)—or of the feedback mechanisms involved in the brain’s hemodynamic control. Changes in the normal feedback mechanisms may include tissue acidosis, extracellular potassium increase, or alterations in cerebral neural pathways. Neurotransmitters can reach vasoactive levels in perivascular CSF as a result of synaptic overflow during neuronal activation or in pathologic conditions.
Neurogenic Regulation
A major difference between other systemic circulations and the cerebral circulation is the relative lack of humoral and autonomic control on normal cerebrovascular tone. Hence, a maximal stimulation of the sympathetic or parasympathetic nerves alters CBF only slightly (41). Furthermore, there is considerable evidence that indicates the existence of age-related differences in cerebral resistance vessels to neural stimuli. For example, both in vivo and in vitro, cerebrovascular constrictor responses to noradrenaline or electrical transmural stimuli are greater in fetal and neonatal animals than in adult animals. The mechanism for the age-related decrease is unclear, but could be the result of such factors as loss of number or affinity of α-adrenergic receptors with development. However, changes in cerebrovascular sensitivity to α-adrenergic stimuli may not occur with age in all species. Electrical or reflex activation of sympathetic nerves reduces CBF in adult rabbits. Sympathetic stimulation may protect the cerebral circulation from hyperemia associated with even modest elevations in arterial blood pressure.
Other Factors Regulating Cerebral Blood Flow
Although cardiac output hardly influences CBF in normal conditions, it may significantly influence flow to ischemic regions (42,43). However, studies examining the possible relationship between changes in cardiac output and CBF have, for the most part, assessed the effect of drugs that increase cardiac output during either normotension or induced hypertension. Improving cerebral perfusion by volume loading is indirectly accomplished by improving blood rheology and directly accomplished by increasing systemic arterial pressure and preventing occult decreases in systemic pressure in hypovolemic patients.
Because blood viscosity is a major determinant of vascular resistance, CBF is inversely related with hematocrit (44). Nevertheless, a continuing controversy questions whether CBF is purely rheologic or a function of changes in oxygen delivery to the tissue (45). Bouma and Muizelaar have claimed that viscosity directly participates in cerebral hemodynamic autoregulation, termed viscosity autoregulation (39).
CEREBRAL FUNCTION
Clinical Examination
Cerebral function can be monitored with instrumentation or assessed clinically. As the discussion of individual monitoring modalities below shows, each monitor offers only a small window into the state of the CNS. Even in combination, current monitors have significant limitations in spatial and/or temporal resolution. A neurologic examination of an alert patient, on the other hand, can comprehensively assess the function of the CNS. Furthermore, it can be repeated as often as needed and requires neither expensive technical equipment nor specialized technologists.
In clinical practice, however, the neurologic examination has important limitations. First, the patient’s clinical status or underlying disease may limit the amount of information obtainable by a clinical examination. Second, the results and, by extension, the utility of a neurologic examination may be constrained by therapeutic interventions that are frequently used in the ICU. For example, medications used for analgesia or sedation will alter the neurologic examination to varying degrees, making differentiation between drug effect and clinical deterioration very difficult. In an intubated patient who is treated with neuromuscular-blocking agents, the only evidence of recurrent generalized seizure activity may be increased ICP, while the postictal alteration of consciousness and the motor manifestations of the seizure go unnoticed. Finally, neurologic evaluations are performed intermittently and by examiners of variable skill, raising problems of reliability and reproducibility. Despite these limitations, the clinical examination forms the cornerstone of the neurologic assessment of ICU patients and typically directs further diagnostic or therapeutic interventions.
While a comprehensive discussion of a clinical neurologic examination is beyond the scope of this chapter, two aspects of the examination that are particularly pertinent to the ICU environment will be discussed in some detail. The first is the assessment of the level of consciousness, because of its ties to patient outcome for many different neurologic diseases. The second is the examination for assessing brain death, not only because it is a graded assessment of brainstem function, but also because it illustrates sources of error that may impact the results of the clinical neurologic examination in the ICU environment in general.
Level of Consciousness: Glasgow Coma Scale
The level of consciousness is typically assessed by the Glasgow coma scale (GCS). Numerical scores are assigned for best responses in the categories of eye opening, motor response, and verbal response (Table 28.2). The GCS was originally described more than 30 years ago for the continuous assessment of patients with TBI after the initial period of stabilization (46). Because its assessment is quick, objective, and relatively reliable (47), and because the resulting score is easily documented and communicated, the GCS has gained widespread use in emergency medicine and critical care patients. It has been incorporated into the Acute Physiology and Chronic Health Evaluation (APACHE) score (48) and the World Federation of Neurosurgical Societies (WFNS) grading of subarachnoid hemorrhage (49).
The level of consciousness is a reflection of the severity of many different disease states, and can be compromised not just by diseases of the CNS, but also at the extremes of a wide variety of other organ dysfunctions common in critical care. Not surprisingly, therefore, the scores from a tool such as the GCS that assesses the level of consciousness may be associated with prognosis and outcome. For the GCS, an association of lower scores with worsened outcome has been shown for TBI (50), subarachnoid hemorrhage (51), brain abscess (52), survival after cardiac arrest (53,54), and septic encephalopathy (55). For example, in TBI, a GCS score greater than 7 suggests a 90% likelihood of an outcome of moderate disability or better, whereas a score less than 7 suggests an increased risk of death or persistent vegetative state that approaches 60% to 90% for a GCS score of 3 (50,56,57). New data suggest that, at least in children, early decompressive craniectomy significantly increases the likelihood of better outcome (only mild disability) (58). In aneurysmal subarachnoid hemorrhage, a GCS score less than 13 after initial treatment of increased ICP (i.e., WFNS grade 4 or 5) corresponds to a 60% to 90% chance of a poor functional outcome or death, whereas such outcomes only affect 14% of patients whose level of consciousness is unaffected (GCS 15, WFNS grade 1 or 2) (51).
TABLE 28.2 Glasgow Coma Scale | |||
![]() |
Despite its widespread use and appeal, the GCS has several important limitations, even if applied correctly. One is the information loss inherent in reducing a graded assessment of three responses into a single number. The second is that mechanical problems such as swelling and endotracheal intubation may prevent proper assessment of eye opening and verbal response. In this setting, some clinicians assign the lowest component score, whereas others try to infer the “true” score from related neurologic findings, and still others add the subscript “T” to indicate an intubated patient. Third, sedatives and neuromuscular-blocking agents affect the GCS score upon repeated assessment. Finally, although the degree of brainstem involvement may reflect the severity of coma, the GCS provides limited information about brainstem function.
Determination of Brain Death
The determination of brain death for purposes of organ donation or withdrawal of life support is an area that has brought both the merits and the limitations of the neurologic examination into clear focus. Because the clinical determination of brain death requires a comprehensive and methodical assessment of the patient (59), its steps may serve as a guide to the neurologic examination of a comatose patient. An algorithm for the determination of brain death is shown in Figure 28.6. Given the gravity of the “therapeutic” consequences of the diagnosis of brain death, a prerequisite to its determination is a clinical picture, typically supported by imaging studies, that is consistent with the occurrence of brain death.

Figure 28.6. Evaluation of a patient in a coma.
TABLE 28.3 Neurologic States Resembling Brain Death | ||
![]() |
The first step in the neurologic examination for the determination of brain death is the determination of coma (i.e., lack of responsiveness to external stimuli due to unconsciousness as discussed above). Motor responses elicited by the examination need to be differentiated from spontaneous movements during the examination. The latter are typically brief, slow movements that originate from the spinal cord and do not become integrated into decerebrate or decorticate responses. Only rarely are they reproducible upon repeat testing. Reproducible partial eye opening that failed to reveal the iris has been described in response to a peripheral painful stimulus in a patient who fulfilled clinical criteria of brain death (60). Conditions that may confound the clinical diagnosis of brain death are listed in Table 28.3. In addition to considering such confounding conditions, the diagnosis of brain death should be consistent with imaging studies and/or the overall clinical picture before the formal determination of brain death is considered.
The next step in the neurologic examination is the assessment of brainstem function. As in the assessment of the level of consciousness, direct trauma to either afferent or efferent structures needs to be considered before any of the tests of brainstem function are interpreted as negative. Typical tests, their afferent and efferent pathways, and potentially interfering clinical conditions are summarized in Table 28.4.
TABLE 28.4 Clinical Examination of the Brainstem during Evaluation for Brain Death | |||
![]() |
To complete the diagnosis of brain death, an apnea test is performed to test the response to an acute decrease in the pH of CSF due to hypercarbia. Hypercarbia is induced by disconnecting mechanical ventilation while continued oxygenation is assured by both preoxygenation and apneic oxygenation. Absence of respiratory movements at an arterial PCO2 of 60 mmHg or after an increase in PCO2 of 20 mmHg is consistent with brain death. Apnea testing may be complicated by arterial hypotension due to loss of arterial and autonomic tone (61). While such hypotension corroborates the diagnosis of brain death, it makes the hemodynamic stability required for apnea testing difficult to attain. The apnea test may trigger movement responses, which reflect residual spinal activity (62).
Once all these criteria for brain death are met, an observation period followed by repeat assessment or a confirmatory test is used to reach a final diagnosis (see Fig. 28.6). Cerebral angiography is the gold standard among confirmatory tests. Contrast media is injected into the aortic arch and distributes to the external carotid circulation, whereas the internal carotid and vertebral arteries fill only to the level of the skull base and atlanto-occipital junction, respectively. Similar findings can be obtained with MR angiography or with single photon emission CT 99mTc-HMPAO. Electroencephalography (EEG) and transcranial Doppler (TCD) are also frequently used as confirmatory tests. Their role will be discussed in greater detail below.
Electrophysiologic Techniques
Neurophysiologic function testing has been used for more than 20 years as a diagnostic/prognostic tool in the ICU (63–66). Snapshots of function of different parts of the nervous system have been used to predict the most likely long-term function of the nervous system as a whole. This information helps the intensivist determine whether continued aggressive intensive care is appropriate given the patient’s most likely long-term neurologic outcome. To a much lesser extent, neurophysiologic testing modalities have been used as continuous monitors of neurologic function in the patient who cannot be assessed neurologically, primarily because of the need for sedation (67–69). There are two main modalities of neurophysiologic function testing used in the intensive care unit: EEG and evoked potentials (EPs). For each modality, the theoretical basis for use and utility will be reviewed.
Electroencephalography
To understand how EEG can be used in the ICU, the clinician must first understand how scalp-recorded EEG is produced and what factors may affect the recordings. EEG activity is generated by neurons in the pyramidal layer of the cerebral cortex. The scalp-recorded EEG is produced by a summation of excitatory and inhibitory postsynaptic potentials (EPSPs and IPSPs), not actual cellular depolarization. EPSPs and IPSPs are produced by the spontaneous release of small packets of excitatory or inhibitory neurotransmitters from a nerve terminal that produce only very small changes in the postsynaptic membrane potential, insufficient to cause depolarization. As a result, the amplitude (voltage) of EEG electrical activity is much smaller than the electrocardiogram, ranging from less than 5 μV in the elderly to over 100 μV in the teenager. As a result, the EEG signal cannot be recorded remotely from the generator site, and practically speaking, EEG activity recorded from a single electrode only reflects cortical activity directly beneath the recording site. In addition, because the EEG signal is so small, poor electrode contact with the scalp may result in significant loss of signal.
Maintenance of ion fluxes associated with the production of the EEG is an energy-requiring process. Pharmacologic total suppression of the EEG will result in a 50% to 60% decrease in CMRO2 (70,71). The decrease in oxygen requirement parallels the suppression of the EEG in cases of lesser suppression. An EEG that is merely slowed pharmacologically will be associated with a higher CMRO2 than an EEG that is totally suppressed or flat.
The EEG is organized spatially and temporally, but patterns of organization are much more difficult for the clinician to recognize, primarily because few clinicians have significant experience with normal EEG patterns, pathologic EEG patterns, or drug-induced EEG patterns. EEG patterns are described primarily in terms of frequency (how fast voltage oscillations occur) and amplitude (size or voltage). Slower frequency ranges include δ (3 Hz or slower) and θ (3.5 to 7.5 Hz). These frequencies are not seen in the normal awake adult but are commonly seen in the naturally asleep adult or in the adult who is receiving therapeutic doses of sedative-hypnotic and/or analgesic drugs. Faster frequency ranges include α (8 to 13 Hz) and β (>13 Hz). Alpha frequencies (8 to 13 Hz) tend to be present on the posterior part of the head and are most prominent with the eyes closed. Alpha activity disappears with attention and concentration, replaced with faster β activity. Beta frequencies are commonly seen more toward the front of the head and are associated with increased “function” of a particular part of the brain. In the neurologically abnormal patient, θ and δ frequencies may be focal, associated with a specific loss of function, or more global, associated with generalized neurologic dysfunction. Generally, the more severe the neurologic damage/dysfunction, the slower the recorded EEG activity will be. For example, a patient with a receptive and expressive aphasia will likely demonstrate EEG slowing (θ and δ waves) over the dominant temporal lobe.
Sedative-hypnotic drugs produce a change in neurologic function that is likewise paralleled by EEG changes. The EEG changes associated with sedative-hypnotic drugs are predictable, related both to the drug used and the dosage of drug given. The vast majority of sedative-hypnotic drugs used in the ICU will produce identical, dose-related changes in the EEG. Table 28.5 shows EEG pattern changes associated with most drugs that would be used in the ICU environment. Dexmedetomidine sedation produces EEG patterns indicating that patients are more sedated than they actually are. Even a low-dose infusion produces a combination of slow delta oscillations with bursting 9- to 15-Hz spindles (72). Combinations of drugs, of course, will have different effects than when either drug is used alone. Specific data regarding the effect of combinations of drugs is limited and beyond the scope of this chapter. However, in general, both sedative and analgesic drugs will increase the primary effect of the drug being used in the higher dose as well as add effects of their own.
In summary, the scalp-recorded EEG reflects the function of closely underlying neuronal tissue. These functions may be altered by neurologic damage, pharmacologic means, normal changes in function associated with changes in alertness or sleep, or any combination of these factors. Thus, whether the EEG is used as a monitor or a diagnostic/prognostic tool, interpretation of data without a thorough knowledge of all factors that could influence recordings is not possible.
Diagnostic Electroencephalography in the Intensive Care Unit
Diagnostic EEG studies or EEG monitoring in the ICU is done primarily for one of three purposes: Brain death determination, monitoring for evidence of seizure activity or cerebral ischemia, and determination of drug effect for the purposes of titrating sedative and analgesic drugs or control of ICP.
Criteria for brain death vary from state to state, but in most states, a 16- to 32-channel isoelectric EEG on two consecutive recordings at least 24 hours apart can provide strong corroborating evidence for cessation of brain function (see Fig. 28.6). Because other factors affecting the EEG can produce an isoelectric EEG in the absence of brain death, the EEG cannot be used as the sole evaluation for brain death. Although it is likely that drug levels (see Table 28.5) will decline significantly over a 24-hour period, patients with massive drug overdose or impaired metabolic pathways may show an isoelectric EEG for much longer than 24 or 48 hours. In these cases, the neurologic examination may also not be useful because high drug levels may suppress even the most resistant reflex responses. Fortunately, other diagnostic testing methods, including other electrophysiologic and nonelectrophysiologic methods, may be helpful. EPs (see below), for example, are more resistant to drug effects than the EEG and can frequently be used to demonstrate brainstem and cortical function, even in the face of an isoelectric EEG (73,74). In addition, an EEG recorded immediately after cardiac arrest may show an isoelectric pattern that subsequently recovers (75). Cortical EPs have also been demonstrated to be more reliable in assessing neurologic function immediately after an ischemic/anoxic insult (75). In summary, a scalp-recorded, 16- to 32-channel EEG is a helpful adjunct to the diagnosis of brain death, provided all other factors influencing the EEG are understood and controlled.
TABLE 28.5 Sedative-Hypnotic and Analgesic Drugs and the Electroencephalogram (EEG) | |||
![]() |
Continuous EEG monitoring in the ICU or, alternatively, sequential diagnostic EEG studies, have been described for detection of nonconvulsive seizure (NCS) activity (or seizure activity in the pharmacologically paralyzed patient) and for detection of cerebral ischemia (67–69,76–78). This type of monitoring requires multiple channels of information to obtain adequate monitoring coverage of the entire brain. A highly trained technologist observes the patient simultaneously with the EEG recording and operates the equipment and maintains recording electrodes during nursing care that will commonly dislodge them. The technologist also provides real-time neurophysiologic data to the clinicians caring for the patient. Processed EEG algorithms have been developed to facilitate detection of ischemia epileptiform and frank seizure activity during continuous EEG monitoring (79,80). Although the technology shows promise even in the hands of nonneurophysiologists (81), this technique has not yet evolved enough to eliminate the need for an on-site technologist with monitoring experience.
Continuous EEG monitoring in the ICU has demonstrated that NCSs are much more common than previously thought (77,78,82). NCSs have been reported following neurosurgical procedures, subarachnoid hemorrhage, CNS infection, head injury, and other conditions. In addition, there is evidence using neuron-specific enolase as a marker of neurologic injury that NCSs may produce neurologic damage and that seizure duration and time to diagnosis are significantly related to the extent of damage and long-term outcome. Without continuous EEG monitoring, NCSs cannot be detected, as they are not consistently and specifically associated with other findings such as hypertension and tachycardia (82).
The personnel and fiscal costs of continuous EEG monitoring have made it unfeasible except in the larger neurologic and neurosurgical ICUs, where many patients with conditions amenable to continuous monitoring require care (76). In addition, very little outcome data exist to demonstrate that such monitoring is overall cost effective. When considering real-time neurologic monitoring in the patient whose neurologic examination cannot be assessed, much work needs to be done to determine how continuous EEG monitoring will mesh with other neurologic monitoring modalities such as ICP, CBF, brain tissue pO2 monitoring, TCD, and microdialysis monitoring. Theoretically and based on limited clinical data (76–81), there is much promise for continuous EEG monitoring when used as a part of a multimodality neurologic monitoring program.
Monitoring of Sedation by Processed Electroencephalogy
The use of the EEG to monitor the depth of sedation in patients in the ICU has been described extensively in the literature, and nearly all techniques utilize processed EEG rather than the unprocessed analog signal. Drug effect monitoring is generally accomplished using one or two channels of EEG information, generally recorded over the frontopolar region of the cerebral cortex. This location is chosen because application of surface recording electrodes is easy in this location (no hair) and most devices designed for this purpose have been validated using frontopolar recording locations. Usage of this smaller number of channels is based on the assumption that the drug effect will be similar in all areas of the brain. This assumption is generally valid except in the case of a patient with focal brain damage. In areas of damage, the drug effect will generally be greater than usual and must be interpreted in light of the abnormal baseline recording. None of the commercially available devices for monitoring drug effects on the EEG has been calibrated or validated appropriately for monitoring drug effects in the patient with the abnormal EEG, and relatively limited information is available on the use of EEG to monitor drug effects in neurologically damaged patients (83–86).
EEG drug effect monitoring is used most commonly for titrating sedative drugs, particularly in the pharmacologically paralyzed patient, but also for titration of barbiturate drugs or propofol used to control ICP (67–69,87,88). Devices used to monitor the drug effect utilize unprocessed, raw analog EEG in a fashion similar to ECG monitoring in the ICU or utilize one of three signal processing techniques: Power spectrum analysis, bispectral analysis (BIS), or EEG entropy analysis. Examples of commercial monitors include the BIS, the patient state index, SEDline, and entropy. Although the BIS monitor has been used and studied most widely among these monitors, the concepts discussed below should apply to other EEG-based monitors of sedation as well.
BIS (Aspect Medical Systems, Inc., Natick, MA) monitoring has been used in the intensive care setting to guide dosing of sedatives and reassure clinicians that paralyzed or agitated patients are amnestic but not excessively sedated (89). The BIS monitor processes EEG signals that are recorded from a self-adhesive electrode strip placed on the forehead. It calculates and displays a BIS value, a dimensionless number ranging from 0 to 100 that is derived from highly processed EEG data that includes EEG power, frequency, and bicoherence (90). Low BIS numbers indicate strong relationships among the EEG frequencies and reflect a condition consistent with a deep hypnotic state (Table 28.6). This relationship is valid despite the effects of age and infirmity on sensitivity to sedation (91,92).
Despite its obvious clinical utility, the aspects of imperfect performance of the BIS monitor are well known. For example, the BIS can decrease to numbers (20 to 50) consistent with deep general anesthesia during natural sleep without sedation (93). Moreover, although memory is less likely to form at lower BIS values, memory has been demonstrated even at a BIS in a range (40 to 60) associated with general anesthesia (94). Additionally, artifact from electromyographic, electro-oculographic (95), or pacemaker generators (96) can produce significant but spurious BIS increases (from 50s to 80s), although the algorithm has been improved in the last decade to reduce the effects of such artifacts. This finding raises the possibility of overdosing nonrelaxed or paced patients when attempting to maintain a given BIS range. BIS values can also be driven higher by medications that are CNS stimulants, such as ketamine or methylphenidate (97). Dexmedetomidine, as discussed earlier, may produce EEG patterns that will result in lower BIS values at a comparable level of sedation (71,95). In such cases, the BIS may not reflect the level of hypnosis or sedation experienced by the patient. Therefore, when the sedative dosages required to achieve a desired BIS range exceed normal expectations, the possibility of an artifactual interference deserves consideration.
TABLE 28.6 Clinical Condition Expected with Bispectral Index Values | |
![]() |
Perhaps the most significant issue with BIS or other monitors of cortical anesthetic drug effect are their inability to differentiate deep sedation from cerebral ischemia. Both conditions cause loss of higher frequency EEG waves (α and β slowing and δ and θ wave intrusion) and, in extreme states, both can produce burst suppression or isoelectric EEG patterns with a low BIS. When O2 delivery decreases below a level sufficient to meet the CMRO2, electrical function fails and BIS decreases. This may partly explain improved ICU outcomes when the BIS is maintained above 60 (98). Therefore, the determination that sedation is adequate based on having achieved a target BIS value should only be made when one is confident that cerebral perfusion is adequate.
Interpretation of BIS or, for that matter, any EEG-based monitor of sedation is best accomplished when the patient’s pharmacologic support remains stable in the face of changing CPP or, conversely, the CPP remains adequate and stable during pharmacologic adjustments and BIS changes. As a corollary, the BIS can assist with guiding therapy when the adequacy of O2 supply to the CNS is in question (99).
In summary, other than for drug effect monitoring, use of the EEG in the ICU remains relatively limited, primarily because of personnel costs and difficulty in maintaining stable technical conditions for monitoring multiple channels of information. As our understanding of underlying mechanisms for neurologic injury improves, we may be able to learn which monitoring modalities are most useful for a given clinical scenario and which can more specifically target EEG monitoring to a smaller area of the brain. In addition, as computing power continues to improve, signal processing technology will likewise improve, and EEG monitoring equipment that recognizes artifact and self-corrects technical problems may reduce the need for the continuous presence of highly trained personnel to operate the EEG in the ICU environment.
Peripheral Nerve Stimulation
The rate of recovery from neuromuscular-blocking agents depends upon the neuromuscular-blocking agent chosen, its dosing pattern (intermittent or continuous infusion), and numerous patient factors (e.g., pseudocholinesterase deficiency, hepatic or renal dysfunction, induced cytochrome P450 enzyme, organophosphate toxicity, among many others) (100). The suitability for extubation following prolonged neuromuscular blockade has traditionally relied upon functional strength testing, such as an ability to produce a negative inspiratory force or to sustain a head lift. Incomplete patient cooperation caused by sedation or confusion, among other reasons, can adversely affect these tests. Peripheral nerve stimulation (PNS), used for “muscle twitch” testing, or acceleromyography, complements such functional assessments by objectively revealing the condition of the neuromuscular junction, independent of patient participation.
Reliable interpretation of nerve stimulation requires uniform stimulation and placement parameters. Conventional PNS delivers current—adjustable up to 80 mA—in a train-of-four (TOF) series at 2 Hz as double-burst stimulation, as single shocks at 1.0 or 0.1 Hz, or by tetanic stimuli of 50 or 100 Hz. When tolerated, maximal current settings assure the best chance of delivering supra-threshold stimuli and activation of the greatest percentage of motor fibers despite changes of impedance or proximity, as can occur with electrode separation or desiccation, skin cooling, or peripheral edema. TOF and double-burst stimulation patterns do not require comparison to earlier responses for interpretation, and are therefore well suited for use in the ICU setting where recovery of neuromuscular function may take hours to days and may involve assessments by multiple providers.
Muscle twitch testing measures the force of muscle contractions in response to PNS. The ratio of the force between the last and first stimuli in a series (TOF or double-burst stimulation) best defines the percentage of acetylcholine receptors occupied by nondepolarizing neuromuscular-blocking agents in the neuromuscular junction, but is cumbersome to perform (101). Counting the loss of twitches in a TOF is a simpler method for assessing the level of block and has greater bedside utility (Table 28.7). In contrast, the TOF ratio does not change following the administration of a depolarizing neuromuscular-blocking agent such as succinylcholine. When depolarizing neuromuscular-blocking agents are used, the force of contraction diminishes equally across all stimuli and disappears altogether with sufficient dose. If an excessive depolarizing neuromuscular-blocking agent is administered, a prolonged phase II block emerges. TOF responses during a phase II block behave similarly to responses obtained following nondepolarizing neuromuscular-blocking agents.
The peripheral nerve stimulator is attached to a patient using two pre-gelled electrocardiogram electrodes, although needle electrodes can also be used. The electrodes should be placed closely (without the gels touching) to one another over a site where a nerve with motor function lies relatively superficial to the skin. Antegrade nerve conduction is improved if the positive lead is applied to the proximal electrode. The current path between electrodes should not contain the muscle whose movement is being monitored. Separation of electrodes beyond several centimeters increases the probability that the PNS current may depolarize muscle directly, causing movement unrelated to conduction through the neuromuscular junction and thus, misinterpretation of the level of neuromuscular blockade.
TABLE 28.7 Percentage of Neuromuscular Junction Blockade with Nondepolarizing Neuromuscular-Blocking Agents and Corresponding Train-of-Four and Clinical Responses | |
![]() |
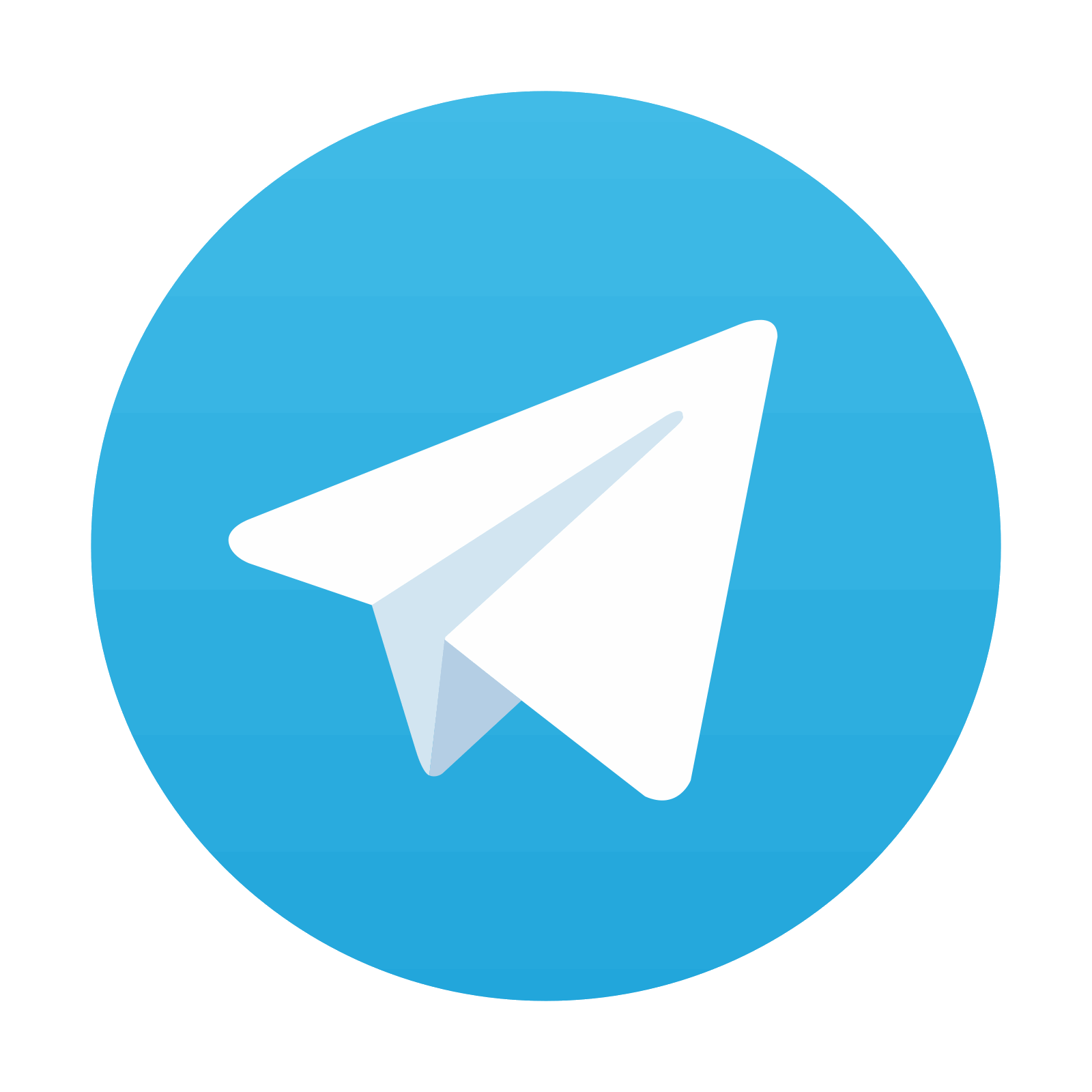
Stay updated, free articles. Join our Telegram channel
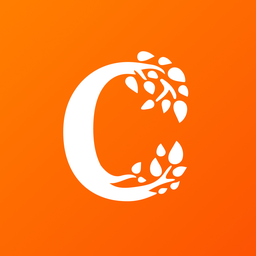
Full access? Get Clinical Tree
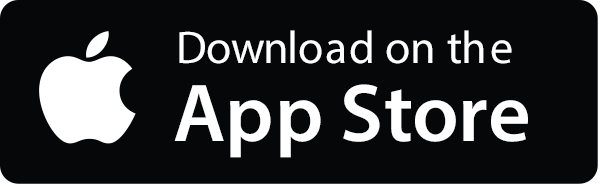
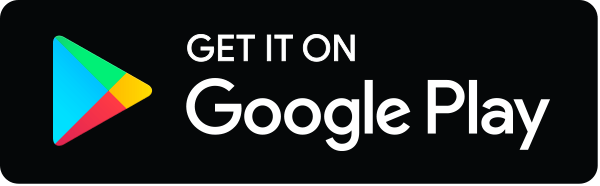