Neurologic Imaging
David J. Michelson
Stephen Ashwal
KEY POINTS








Care for children with critical central nervous system (CNS) injuries has been substantially improved by advances in neuroimaging. Cranial computed tomography (CT) and magnetic resonance imaging (MRI), the two most commonly used techniques, provide images with increasingly specific diagnostic and prognostic anatomical and functional information. This chapter discusses these techniques and their use in common pediatric critical care applications.
TECHNIQUES
Computed Tomography
CT images, generated by the collection and analysis of x-ray beams as they are sent through tissues from multiple angles, are more than adequate for the identification of surgically remediable forms of acute neuropathology, such as fractures, tumors, large hemorrhages, and obstructive hydrocephalus. One major factor in favor of CT in comparison with MRI is that it can be quickly and easily obtained. Helical (spiral) scanners have so substantially shortened the acquisition times required
for high-quality images that sedation for CT is unnecessary in the vast majority of infants and children who can refrain from moving briefly or who can be lightly restrained (1). One of the drawbacks of CT is radiation exposure, particularly of concern for young patients and those who require repeated studies, given the known dose-related risk of radiation-associated neoplasia (2) and possible risk of developmental impairment (3). Considering this, alternative studies, such as ultrasound, MRI, or CT of lower resolution (and thus lower radiation dose) may be preferable. Also, while the radiodense, iodinated contrast agents used in CT scanning are well tolerated by most children, acute allergic reactions can occur, particularly in older children and in children with a history of asthma. Portable CT machines have been developed that make it possible to safely acquire images of reasonable resolution in patients who might otherwise be too unstable for transport to a fixed scanner. Studies have shown clinical efficacy and cost-effectiveness for use of such devices in patients who are receiving critical monitoring or therapies in NICU, PICU, ER, and operating room settings (4).

Conventional Anatomic Imaging
Axial images of the head are routinely obtained with a slice thickness of 5 mm, although thinner slices can be obtained if greater detail is required, and images can be reformatted into other planes or into three dimensions. The brightness of each voxel (a pixel representing a three-dimensional [3-D] volume) on a CT image reflects tissue density. Fatty tissue, water, and air appear dark; soft tissues appear as intermediate shades of gray; and mineralized bone, concentrated blood, iodinated contrast, and metallic objects appear bright. CT is limited by streaking artifacts in areas around metal prostheses, fragments (such as dental fillings or gunshot pellets), and dense bone (such as the temporal petrous bones in the posterior fossa). Areas of abnormal signal on CT can be characterized as hypodense, isodense, or hyperdense, relative to the normal neural tissue. The differential for hypodense parenchymal lesions includes edema, infarction, neoplasia, demyelination, inflammation, and cyst formation. Hyperdensity is found in areas of contrast enhancement, hemorrhage, calcification, and hypercellularity. Abnormal calcification can be seen with congenital or chronic infections, tumors and hamartomas, abnormal blood vessels, areas of ischemia, metabolic disorders, and endocrine disorders (5). Calcification of subependymal nodules in a patient with tuberous sclerosis complex is shown in Figure 59.1. Isodense lesions may be recognizable by their replacement of, or effect on, normal structures, unless they show abnormal contrast enhancement.
![]() FIGURE 59.1. Four-month-old boy presenting with infantile spasms. Axial CT shows multiple bright nodules along the surface of the lateral ventricles due to subependymal hamartomas associated with tuberous sclerosis complex. |
CT Angiography
CT angiography allows the vascular anatomy of the brain to be imaged fairly well by high-resolution CT scanning that is closely timed with an intravenous bolus of an iodinated contrast agent. A test bolus is initially given so as to determine the time delay required to capture images within either the arterial or the venous phase, depending on the study desired. Thinly cut source images can be reformatted into 2- and 3-D images that are nearly as detailed as MR angiograms. As with anatomic imaging, the choice of CT depends on the constraints of radiation exposure, contrast sensitivity, and timing. CT angiography is faster than MR angiography and can usually be done without sedation in older children.
Magnetic Resonance Imaging
MR images are generated by analysis of signals produced by hydrogen nuclei of molecules in varying tissues as the spins of the nuclei are aligned in a strong external magnetic field and then perturbed by radiofrequency pulses. MRI is the modality
of choice when resolution of fine anatomic detail is desired. Moreover, this technology also offers several specialized sequences that highlight metabolic changes within the brain and are applicable to a wide variety of diagnostic situations. MRI is also the imaging test of choice for spinal cord injury when neurologic deficits are present, as CT images of the spine,
while superior for visualizing fractures and dislocations, do
not allow for adequate assessment of the cord. MRI also allows for far more precise imaging of posterior fossa structures, as it is not subject to the artifact produced by the dense temporal petrous bones on CT.



The principal drawback of MR imaging is the long acquisition time, which frequently necessitates procedural sedation for most children less than 8 years old and for some older children. An audiovisual system within the scanner greatly reduces the need for sedation in children over 3 years old. When intravenous propofol is used for sedation, fractional inspired concentrations of supplemental O2 above 0.60 can cause artifactual cerebrospinal fluid (CSF) hyperintensity within the sulci and cisterns that can be mistaken for subarachnoid hemorrhage (6). Another drawback of MRI is the incompatibility of ferromagnetic materials with the strong electromagnetic field within the imaging suite. Plastic and aluminum MR-compatible monitors, ventilators, and anesthesia machines are available, but patients with metallic bullet fragments or implants, including cardiac pacemakers and neurostimulators, may not be able to undergo MRI safely. Information regarding the MRI compatibility of implanted medical devices is maintained at http://www.MRIsafety.com (7). Even metallic objects that pose no safety risk (tracheostomy tubes with spiral metal reinforcement) may create artifacts and distortions in the surrounding tissues. Gadolinium contrast for MRI studies is safe for most patients but does pose a risk of nephrogenic systemic fibrosis for patients with severely impaired renal function. Detailed guidelines regarding the safe use of contrast agents for CT and MRI are published online by the American College of Radiology (http://www.acr.org/quality-safety/resources/contrast-manual).
Conventional Anatomic Imaging
As with CT, MR images are gray-scale maps, with the shade of each voxel reflecting the composition of the tissue it represents. The shade of a voxel on an MR image is referred to as its intensity, rather than its density, because it is determined by factors beyond proton density, including proton mobility (T1 relaxation) and local magnetic effects (T2 relaxation). Various sequences, such as spin echo (SE), gradient-recalled echo (GRE), and fluid-attenuated inversion recovery (FLAIR), use programmed pulses of radiofrequencies and gradient magnetic fields to produce images that highlight T1 or T2 signal effects. On T1-weighted images, fat, methemoglobin, and gadoliniumcontaining contrast agents appear bright (or hyperintense), while CSF, muscle, deoxyhemoglobin, and hemosiderin appear dark. On T2-weighted images, CSF, edema, extracellular methemoglobin, and areas of hypercellularity, infarction, and demyelination appear bright, while muscle, cortical bone, deoxyhemoglobin, and hemosiderin appear dark.
Routine brain imaging in most institutions begins with a sagittal T1-weighted sequence that serves as a localizer for subsequent sequences and allows evaluation of the corpus callosum, pituitary gland, cerebellar vermis, and other midline structures. Axial T1- and T2-weighted images are also routinely acquired, although coronal images may be particularly helpful for investigation of the cerebellum, temporal lobes, and skull base. FLAIR sequences have improved sensitivity in older children for areas of abnormal intracranial T2 brightness in close proximity to CSF-filled spaces, such as the ventricles and sulci, which are bright on conventional T2 studies but dark on FLAIR images. High-resolution volumetric 3-D T1-weighted scans are particularly useful for patients who undergo evaluation of developmental abnormalities and patients undergoing surgical planning.
Diffusion-weighted Imaging
The diffusion of water molecules can be measured along any 2-D plane to which a strong magnetic field gradient has been applied. Diffusion reflects random (Brownian) motion, capillary flow, and transcellular active transport. In standard diffusion-weighted imaging (DWI), bright areas reflect decreased or restricted movement of water along the studied plane. The apparent diffusion coefficient (ADC) map is an additional
sequence that summarizes the diffusion in all three dimensions with the coloring reversed, such that areas in which all water movement is restricted appear dark. DWI is particularly useful in the early evaluation of ischemia, as diffusion restriction becomes apparent within minutes after cytotoxic injury is sustained (8). DWI is also used in the evaluation of brain tumors, helping to distinguish cystic tumors from epidermoid tumors and recurrent tumors from areas of peritumoral edema. Diffusion restriction is also seen within cerebral abscesses and empyemas, and this methodology is helpful in the evaluation of an intracranial cystic lesion that may be an abscess but that cannot otherwise be distinguished
from a tumor with central necrosis (9).
sequence that summarizes the diffusion in all three dimensions with the coloring reversed, such that areas in which all water movement is restricted appear dark. DWI is particularly useful in the early evaluation of ischemia, as diffusion restriction becomes apparent within minutes after cytotoxic injury is sustained (8). DWI is also used in the evaluation of brain tumors, helping to distinguish cystic tumors from epidermoid tumors and recurrent tumors from areas of peritumoral edema. Diffusion restriction is also seen within cerebral abscesses and empyemas, and this methodology is helpful in the evaluation of an intracranial cystic lesion that may be an abscess but that cannot otherwise be distinguished

![]() FIGURE 59.2. Four-year-old boy with a history of complex congenital heart disease (including a double outlet right ventricle) who underwent cardiac bypass for an arterial switch procedure. Diffusely scattered punctate hemorrhages at the cortical-subcortical junction are visible on MRI using the SWI sequence (A) that would not have been identified using T1-weighted (B) and FLAIR (C) sequences. |
Susceptibility-weighted Imaging
Deoxygenated blood and certain blood breakdown products are weakly paramagnetic. The artifacts that these materials produce on T2-weighted MR sequences can be exploited in the evaluation of patients for vascular abnormalities and small hemorrhages. Gradient echo T2-weighted imaging has been used for this purpose for some time but is not nearly as sensitive as the increasingly available susceptibility-weighted imaging (SWI) which makes these same paramagnetic artifacts far
more apparent (10) (Fig. 59.2). SWI can also potentially show areas of cerebral ischemia, with more prominent (darker)
veins from increased deoxyhemoglobin concentrations due to greater oxygen extraction in areas with slowed or diminished blood flow (11).

veins from increased deoxyhemoglobin concentrations due to greater oxygen extraction in areas with slowed or diminished blood flow (11).
Diffusion Tensor Imaging (DTI)
Because water will diffuse more freely parallel to white matter fiber tracts, DWI using multiple planes can be used to create color-coded maps of the fiber tracts and to allow visualization of tract disruption by mass lesions, traumatic shearing, and ischemic injury. These images are sometimes called fractional anisotropy (FA) maps, as each voxel of the image is assigned a color based on the portion of its water diffusion that is directional (anisotropic) as opposed to free to diffuse equally in all directions (isotropic) (12). The direction of the diffusivity of each voxel can also be represented as a sum of vectors which are described by their eigenvalues (length) and eigenvectors (directions). The diffusivity of each voxel can be summarized graphically by an ellipsoid with more or less radial (perpendicular) and axial (parallel) diffusivity. Clinical applications currently include presurgical planning for resection of brain tumors and epileptic regions of cortex, although some studies show a benefit in the identification of subtle cortical and subcortical abnormalities in patients with medically intractable epilepsy (13) (Fig. 59.3).
MR Angiography/Venography
Reconstructions of the arterial supply and venous drainage of the brain can be obtained from maximum intensity projections (MIPs) from 2-D or 3-D time-of-flight images. The images created by this process represent blood flow better than luminal diameter, however, and can overstate the degree of stenosis present in a vessel. As the process of generating MIP images may also produce artifacts, any suspected abnormality should be confirmed in the source images. Gadolinium infusion is rarely used for MR angiography as higher resolution non-contrast techniques have come into use (14).
MR Spectroscopy
Spectroscopic imaging most often analyzes the signals generated by protons from a number of clinically important neuronal and glial metabolites, including N-acetyl aspartate (NAA), choline and phosphatidylcholine (Cho), creatine and phosphocreatine (Cre), myoinositol (mI), and lactate. Images can be generated using short, medium, or long echo relaxation times. The short echo images demonstrate a wider variety of metabolites and are useful for assessing inborn errors of metabolism (15). Longer echo times provide more accurate quantification of the principal brain metabolites, useful in the assessment of prognosis in the setting of trauma (16), global anoxic injury
(17), or neoplasia (18).

MR Perfusion
A number of MR methods have been developed for measuring cerebral perfusion. Fast imaging during paramagnetic contrast injection provides a purely qualitative picture of blood volume, relative regional blood flow, and perfusion delay (mean transit time). Alternatively, the protons within the blood entering the brain through the carotid arteries can be used as an endogenous contrast via spin labeling, particularly with higher-resolution machines that can deliver higher signal-to-noise ratios (19
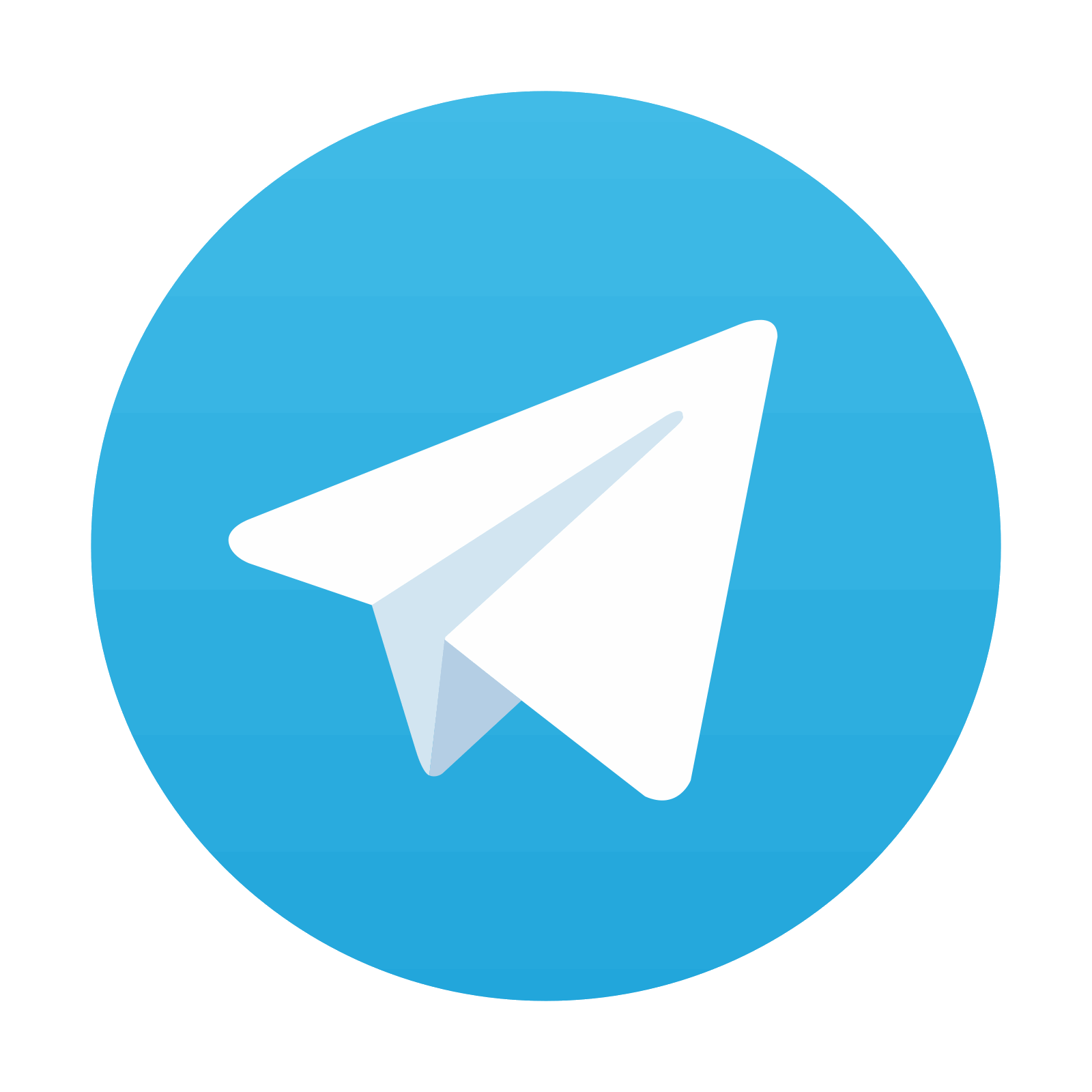
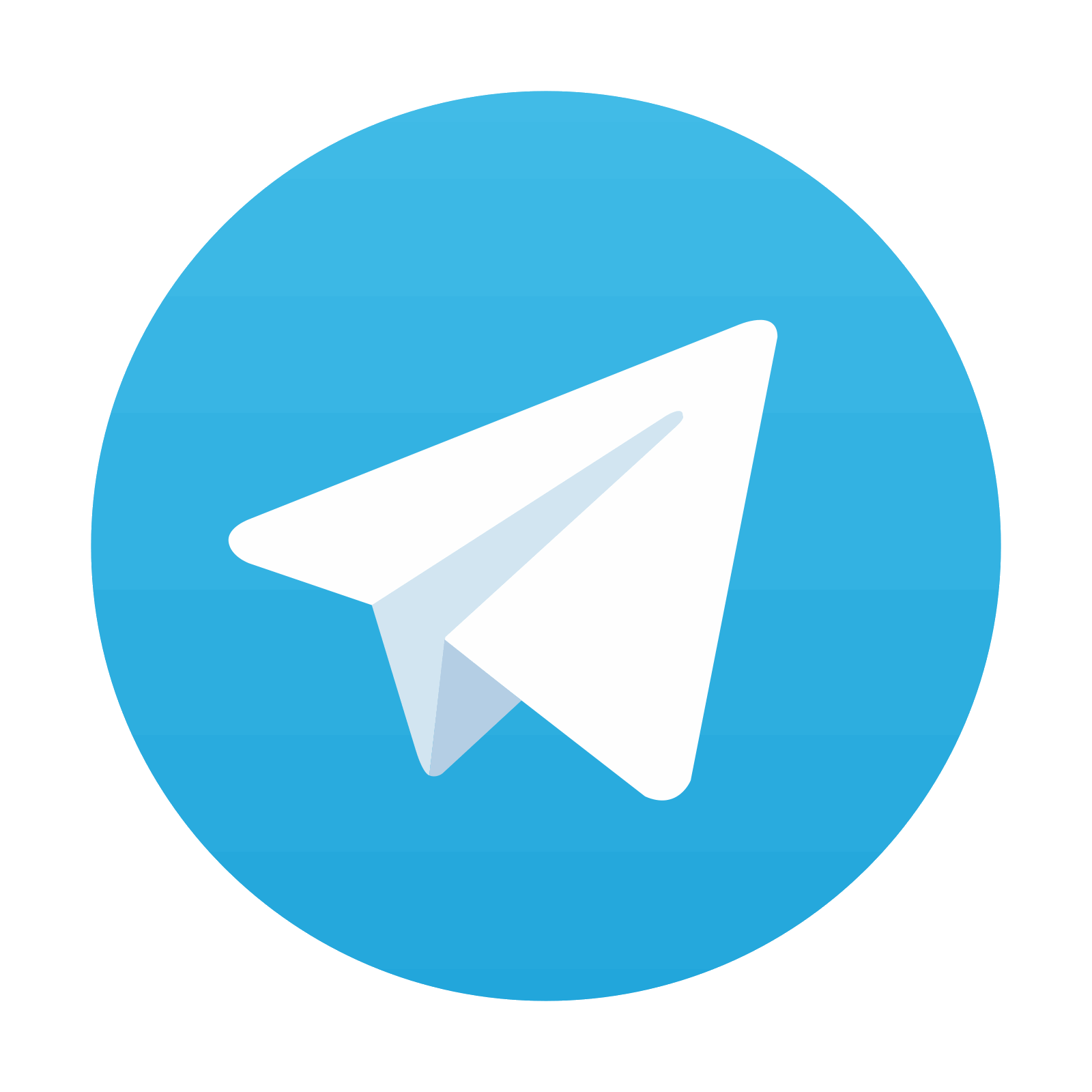
Stay updated, free articles. Join our Telegram channel
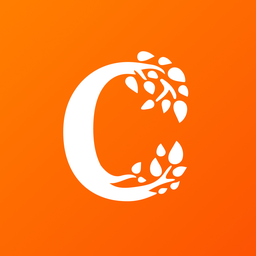
Full access? Get Clinical Tree
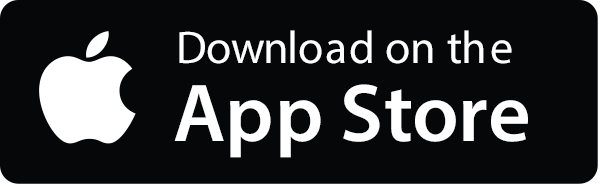
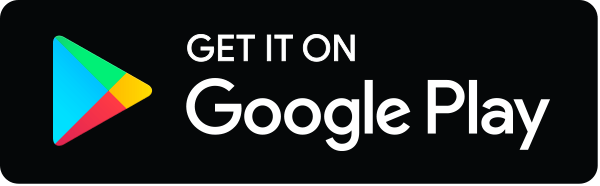
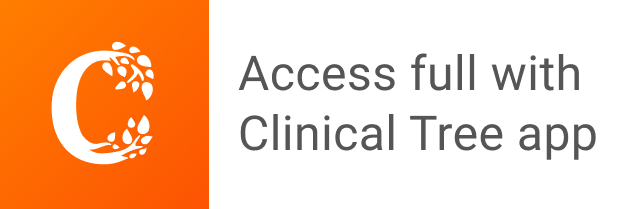