Introduction
Recent advances in technology provide monitoring equipment that helps facilitate complex neurosurgical procedures. New and innovative methods for assessing neurological function in patients undergoing general anesthesia have been developed. The use of new devices and technologies along with the implementation of the new evaluation methods have allowed clinicians to apply this technology in the operating room for continuous monitoring of neurological function.
Neurological Monitoring
Monitoring during neurosurgical procedures requires an advanced understanding of the neuro physiological parameters. Patients with underlying neurological diseases are considered at higher risk for cerebral ischemia when undergoing surgical procedures. Also, patients undergoing neurosurgical procedures tend to have a higher probability of presenting with evidence of past cerebral ischemic events. This risk may be directly associated with the type of neurosurgical procedure performed. It has been established that the use of intraoperative neurophysiological monitoring may improve patient outcome. This also allows physicians to be able to monitor oxygen delivery and to possibly identify intraoperative ischemic events to protect the brain and the spinal cord. Several additional factors increase the likelihood of an undesirable outcome besides the type of surgery, including patient positioning and hemodynamic changes.
Monitoring requirements are determined by the type of surgery and the patient’s physical status. In most cases, the American Society of Anesthesiologists’ (ASA) standard monitors are required. Major intracranial and spinal surgeries may require the application of more invasive monitoring techniques, such as the use of an intraarterial line for invasive blood pressure monitoring when strict hemodynamic control is warranted. In less extensive operations, such as ventricular shunt placements, burr-hole biopsies, and minor spinal surgeries, the use of noninvasive blood pressure monitoring techniques may be sufficient. For vascular procedures and in those where major blood loss is possible, added monitoring of central venous pressure (CVP) is recommended. A complete evaluation of each patient and their comorbidities will determine further monitoring requirements. In patients with underlying heart conditions, monitoring of additional cardiovascular parameters, such as pulmonary artery pressures, may be warranted. The selection of placement of a PAC catheter or a central line must be performed to eliminate the possibility of early intervention and treatment in cases of air emboli.
Core temperature monitoring is also recommended during anesthesia. For nonneurosurgical procedures, there are several locations to which the probe may be applied. The most practical site is often the esophagus. An esophageal temperature probe enables the anesthesiologist to monitor not only the core temperature, but also the heart sounds, if the probe happens to include an esophageal stethoscope. In neurosurgical procedures, the esophageal probe may be replaced by a Foley catheter with an incorporated temperature probe. In patients with high urinary output, special attention must be paid to the interference this may cause with the temperature readings.
The effects of neuromuscular blockers should be monitored with a peripheral nerve stimulator to prevent patient movement or coughing. Movement could be catastrophic during neurosurgical procedures.
Patients are often required to be in a head-up or sitting position during neurological procedures, which increases their risk of venous air embolization through open intracranial sinuses. The use of precordial Doppler ultrasonography (PCD) or transesophageal echocardiography (TEE) is advised for the early detection of such complications. It is also possible to use a Bunnegin-Albin catheter to evacuate air from the right heart in these cases.
Monitors Unique to Neurosurgical Anesthesia
The neurophysiologic parameters targeted are usually brain function, blood flow, and metabolism. Monitoring of function is related to the measurement of electrical activity in the brain by means of electroencephalography (EEG), sensory and motor evoked potentials (EPs), and electromyography (EMG). Monitoring of blood flow/pressure is achieved by nitrous oxide wash-in, radioactive xenon clearance, laser Doppler blood flow, and transcranial Doppler sonography. Alternatively, intracranial pressure can be determined by means of intraventricular catheters, fiberoptic intraparenchymal catheters, subarachnoid bolts, and epidural catheters. Lastly, brain metabolism can be measured either through invasive techniques such as placement of intracerebral P o 2 electrodes or noninvasive techniques, such as transcranial cerebral oximetry and jugular venous oximetry. The increased use of these technologies in neuroanesthesia has improved patient care.
Requirements for a Nervous System Monitor
For a monitor of the nervous system to have maximum usefulness, it must meet certain requirements. To determine if monitors are useful to be considered as standard of care, sufficient evidence needs to be provided whether these monitors accurately reflect the intraoperative condition of the patient and affect the outcome.
The ideal monitoring device should be one expected to detect alterations in the parameters under observation and reveal any changes in the function, blood supply, or metabolism of the organs involved. Certain requirements should be considered when using a device for neurological monitoring. The monitors should have minimal interference or noise, be able to detect surgical injuries and provide the possibility of repair, and be used in a continuous manner. Some neurophysiologic monitoring is not yet considered standard of care and although it is not routinely performed, many institutions are applying it as a regular practice for numerous surgical procedures.
EEG Monitoring
The cortical electrical activity or electroencephalographic (EEG) activity of the brain consumes nearly 50% of the total brain oxygen and the remainder is consumed by cellular integrity maintenance. When the oxygen supply to the brain is decreased by either hypoxemia or decreased blood flow, the remaining oxygen will be shifted towards cellular maintenance and away from the electrical activity of the brain. Brain monitoring is crucial in the prevention of ischemic or hypoxic damage and can be broadly categorized into monitoring of function, blood flow, or metabolism. An EEG signal reproduces the electrical activity of the brain, which is generated by the pyramidal cells of the cerebral cortex and corresponds to the sum of excitatory and inhibitory postsynaptic potentials that are generated. Therefore the EEG measures the electrical function of the brain indirectly, and it can also indirectly measure the blood flow and anesthetic effects. The EEG is recorded from needles or surface electrodes that are placed on the scalp and forehead.
The waves that are generated in the EEG are classified based on their frequencies as beta, alpha, theta, and delta. Beta waves are high frequency, low amplitude waves between 13 and 30 Hz, which are most active during the awake state. Alpha waves are of medium frequency, high amplitude waves between 9 and 12 Hz, which are seen in the occipital cortex when the eyes are shut, while awake. Theta waves are low frequency waves between 4 and 8 Hz. Delta waves are very low frequency between 0 and 4 Hz and with low to high amplitude. Delta waves are consistent with depressed function consistent with a coma state, which can be caused by anesthesia, metabolic factors, or hypoxia.
There are several perioperative applications for the EEG in neuroanesthesia. It can identify if there is adequate blood flow reaching the cerebral cortex during surgical or anesthetic procedures that tend to produce a reduction in flow. The EEG can also perioperatively guide the reduction of cerebral metabolism before the induced reduction of blood flow and it can predict neurological outcomes after brain injury. Other uses for the EEG include indentifying consciousness, measuring seizure activity, and indentifying stages of sleep or coma state.
Any procedure that increases the risk for ischemia or hypoxia in the brain would potentially benefit from EEG monitoring. Procedures that involve manipulation of the cerebral vasculature, such as carotid endarterectomy or cerebral aneurysm repair, can benefit by the use of EEG in management and risk reduction of intraoperative stroke. Deliberate metabolic suppression for cerebral protection during procedures can also be guided by EEG monitoring.
EEG recording is done using an electrode arrangement with bipolar recording due to the fact that there is no electrical neutral area. Both electrodes are active and the recorded signal polarity is dependent on the random designation and not the referential electrode. Common mode rejection, the rejection of the electrical signals that are common to both sites in comparison to the third “ground” electrode, is used to minimize the signal from other electrical activities of the body, such as electromyography and electrocardiography.
The gold standard for EEG recording uses a 16-channel recording with 8 channels for each hemisphere. The electrodes are placed using the International 10-20 system. Due to inaccessibility of the head in general and large parts of the scalp in particular during intracranial surgical procedures, the 16-channel EEG is rarely used during operative procedures. Intraoperative monitoring during craniotomy is usually accomplished with a 2- to 4-channel recording, with computer processing to simplify the recording and interpretation. Most algorithms filter out the high-frequency or 30 Hz activity because it is most likely due to artifact or interference. The raw EEG is then separated using Fourier transformation into component waves, which are then grouped based on their frequency spectrum. This allows the raw EEG recorded in time domain to be displayed in frequency domain. The square of the amplitude of the EEG wave—the power spectrum—can be displayed in several ways. Usually, it is displayed as either compressed spectral array or density spectral array, where either the peaks and valleys or the density of the gray scale represent the power of the spectrum. Another method to process the EEG is the periodic analysis, which tracks and plots each wave as a “telephone pole.” The amplitude or power of the wave is represented by the height of the “pole.”
To interpret an EEG properly, it is necessary to remember that an EEG is recording the spontaneous activity of the brain and reflects how “awake” or metabolically active the brain is. Electrical activity in the brain is a process that requires energy that is dependent on certain substrates, such as oxygen or glucose. Depression of EEG activity and characteristic changes in the EEG can be seen with reduction in cerebral blood flow, or oxygen or glucose delivery. During an awake-state, the high-frequency and low-amplitude beta waves are most prominent. There is a transient increase in the beta waves with the onset of ischemia or hypoxia, with eventual development of large amplitude theta and delta waves. With the increase in ischemia or hypoxia, the beta waves begin to disappear and there is an appearance of low amplitude delta waves. This progresses to electrical activity suppression with occasional bursts of activity. The onset of irreversible damage can be seen when there is complete electrical silence with a flat EEG.
Monitoring the EEG intraoperatively for the development of delta waves allows the anesthesiologist to be aware of an increased risk of ischemic damage to the brain. EEG has a poor predictive value for brain damage and lacks specificity as a diagnostic test for irreversible damage even though it is sensitive to the ischemic changes. The probability for irreversible damage is increased when there is quicker onset of ischemic EEG changes.
Metabolic depression caused by anesthetic drugs or increasing depth of anesthesia can also cause EEG changes in frequency and amplitude that are similar to those seen with ischemia or hypoxia, though these are reversible. Certain inhaled and intravenous anesthetics cause specific EEG changes and are usually dose dependent. Also, hypothermia can cause slowing of the EEG. Due to these influences, the complete clinical picture of the patient should be taken into account with the interpretation of the EEG. Regardless of whether it can be caused by anesthesia or by ischemia, the fast EEG activity is transformed into larger, slower waves, with an eventual decrease in the amplitude of the wave and ultimately the wave becoming flat as metabolic activity decreases.
To simplify EEG interpretation, the anesthetic delivery should ideally be “stable” and not changing during the critical surgical portions of the case. Also, any changes in anesthetic delivery should be communicated to the EEG technician.
The EEG can only provide information of the cerebral cortex function, and not much information on the subcortical brain, spinal cord, or the cranial and peripheral nerves.
Transcranial Doppler
Transcranial Doppler sonography (TCD) noninvasively and continuously measures blood flow velocity in the major vessels in the “Circle of Willis” in the brain, and uses those calculations to make determinations on intracranial hemodynamics. Estimates of cerebral blood flow (CBF) are commonly monitored. Blood flow velocity and actual blood flow are not equivalent, but the two are closely related. By measuring through the thin temporal bone, just over the zygomatic arch with a 2-MHz probe, it is possible to measure the velocity of blood flow in the middle cerebral artery (Vmca). Around 75% to 80% of the ipsilateral carotid blood flows through the MCA. Even though the absolute CBF cannot be measured by the TCD, there is strong correlation between flow velocity changes and CBF. The TCD can also be used for a qualitative assessment of the intracranial pressure or cerebral perfusion pressure, the detection of air or particulate emboli, and determination of cerebral autoregulation and CO 2 reactivity. An advantage from this is that the basal cerebral arteries do not constrict or dilate as changes occur in the vascular resistance, carbon dioxide tension, or systemic blood pressure. Also, the middle cerebral artery is not considerably constricted or dilated with the administration of intravenous or inhaled anesthetics. The blood flow recorded by the TCD in the MCA represents the velocity in that particular artery. Actual velocity in any other vessel cannot be determined unless the diameter of that artery is established. Therefore, TCD is not used to quantitatively determine CBF, but to evaluate for relative blood flow changes.
TCD can be used to evaluate for cerebral vasospasm, transient ischemic attack, stroke, SAH, syncope, brain death, head injury, and AVM. There are several clinical situations where the use of intraoperative TCD is applicable. During carotid endarterectomies, the TCD can be used for the detection of reduced MCA flow or microemboli caused by particulates or air. TCD also allows monitoring for downstream flow reductions indicating inadequate collateralization during cross-clamping of the carotid artery, the detection of vasospasm, or monitoring for shunt malfunction that can be caused by kinking or thrombosis. There is evidence that the correlation between flow-velocity changes on TCD and EEG changes is strong. When compared with preclamping baseline velocity, a decrease in Vmca of greater than 60% suggests poor CBF and the need to use an intraluminal shunt. TCD technology can also be used for the diagnosis and treatment of postoperative hyperperfusion syndrome, which is seen when patients have constant elevated flow velocities even after the release of carotid occlusion and develop headaches, with eventual development of cerebral hemorrhage. In these cases, by promptly reducing the blood pressure, the ipsilateral flow velocity is normalized and the symptoms are alleviated. Postoperatively, the TCD should be promptly used when clinically significant symptoms develop. It can also be used to evaluate for a postoperative thrombi or an intimal flap to prevent the progression of a stroke, or during cardiac surgical cardiopulmonary bypass procedures to monitor for symptoms of cerebral hypoperfusion or for cerebral emboli. It is important to remember that paradoxically, an increase in blood flow velocities indicates a decrease in cerebral blood flow in patients with vasospasm and subarachnoid hemorrhage.
The knowledge of the anatomy and physiology of the cerebral circulation, the skill, and the experience of the technician and interpreter are important for the accuracy of the interpretation of the TCD. Care must also be taken during intraoperative procedures so as not to interfere with the surgical team or the surgical field. The surgical field can limit the probe placement and the maintenance of the proper probe placement. Also, the thickness of the temporal bone is dependent on age, race, and gender, which can influence the results of the TCD.
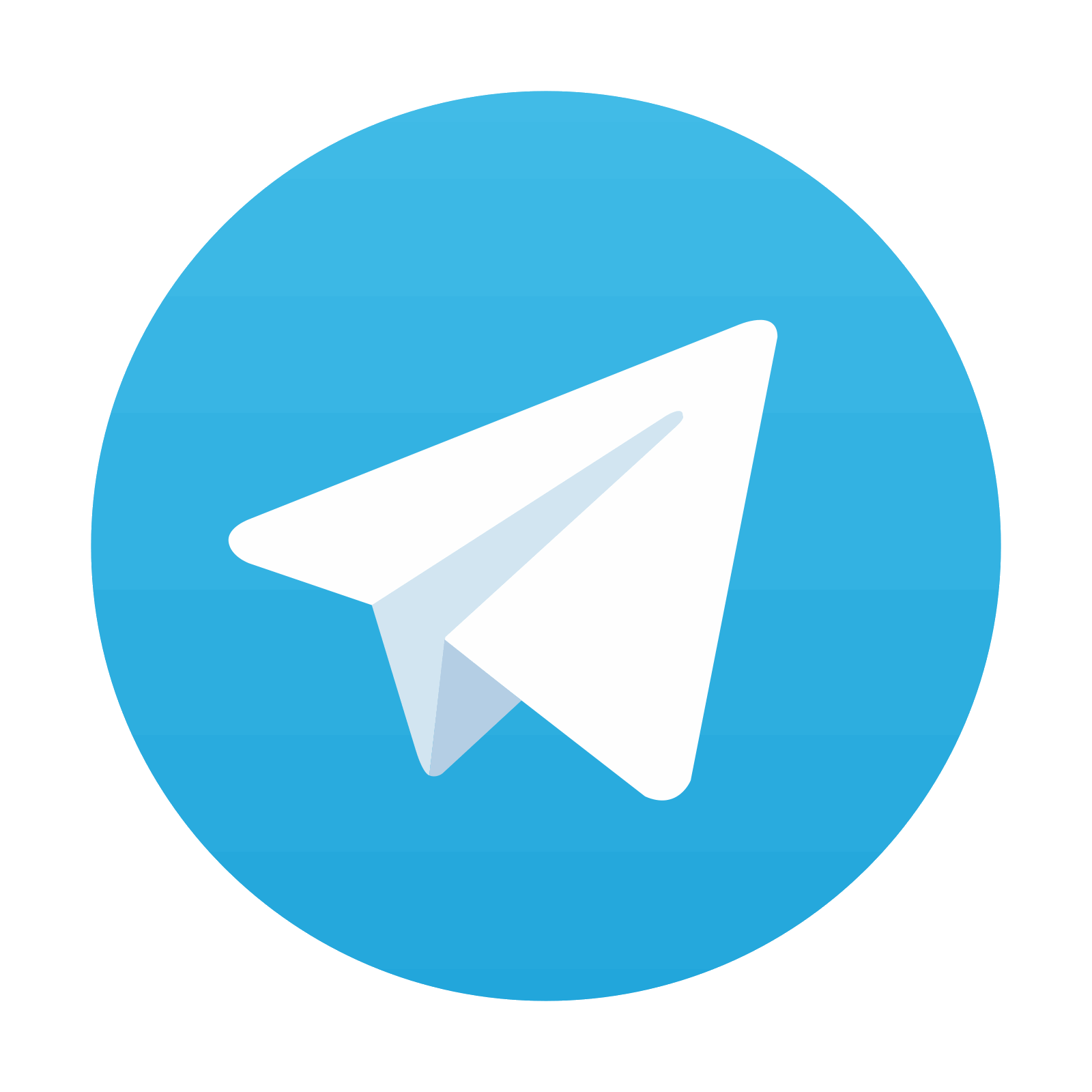
Stay updated, free articles. Join our Telegram channel
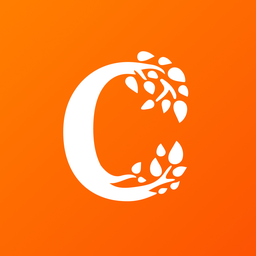
Full access? Get Clinical Tree
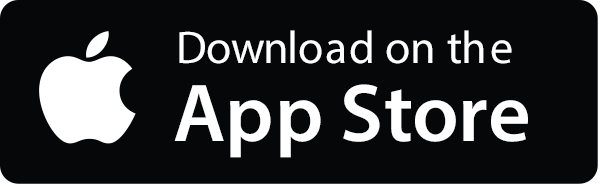
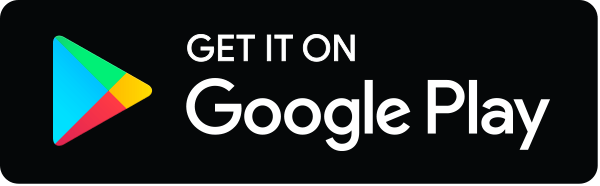