Autoregulation of CBF
The two internal carotid arteries supply ~70 % of CBF, while the vertebral arteries, which form a single midline basilar artery, supply the remaining 30 %. These three arteries form a heptagonal arterial loop known as the circle of Willis that distributes blood throughout the brain (Fig. 29.1). In theory, this redundancy protects the brain from disruptions in blood flow through any one artery. However, a complete and symmetric circle of Willis is present only in ~40 % of the population. Therefore, this necessitates cerebral monitoring (SSEPs, EEG) during many intracranial procedures.


Fig. 29.1
Circle of Willis
The CPP range for autoregulation is often cited as 50–150 mmHg, but significant variation exists across the population. The presence of chronic hypertension may increase these limits to a higher range of pressures, 80–180 mmHg or higher. Thus, a patient with chronic hypertension might experience cerebral ischemia at a CPP of 60 mmHg, whereas an individual without a history of hypertension or intracranial pathology is unlikely to experience ischemia at that pressure. The above two equations imply that CBF is a linear function of CPP, but the flow through healthy brain has multiple intricate and interdependent controls to manipulate cerebral blood flow as listed below (Fig. 29.2):


Fig. 29.2
The impact of MAP, ICP, PaCO2, and PaO2 on CBF. Notice that the brain autoregulates CBF across a wide range of MAP and PaCO2 (MAP mean arterial pressure, ICP intracranial pressure, CBF cerebral blood flow)
1.
Metabolic–perfusion coupling diverts blood flow to metabolically active brain regions so that the oxygen supply matches the demand. This response is robust and remains intact in comatose patients. The mechanism is not well understood, but metabolic by-products (hydrogen ions, ionic gradients, nitric oxide, prostaglandins) likely play a role in producing the vasodilation.
2.
Healthy cerebral vasculature responds to increased MAP with vasoconstriction that reduces cerebral blood volume (CBV) and ICP. The opposite is also true: A decreased MAP leads to vasodilation in an effort to maintain perfusion. Autoregulation alters arteriolar tone to provide a stable CBF over a range of CPP. Autoregulation is impaired to various degrees after stroke, hemorrhage, traumatic brain injury (TBI), and extremes of temperature. Multiple factors play a part in autoregulation—thus, no single target can be manipulated to restore autoregulation.
3.
Autonomic innervation of large cerebral vessels: This theory purports that parasympathetic stimulation increases CBF (vasodilation), while sympathetic activation decreases CBF (vasoconstriction), independent of associated changes in MAP or CPP. Whether this mechanism is clinically relevant is controversial.
4.
PaCO2 reactivity: CBF is directly proportional to PaCO2 between arterial tensions of 20–80 mmHg, with CBF changing 1–2 ml/100 g/min for every mmHg change in PaCO2. Dissolved carbon dioxide diffuses across the blood–brain barrier and alters the pH of the cerebrospinal fluid (CSF). A decrease in the dissolved CO2 (e.g., hypocapnia secondary to hyperventilation) causes CSF alkalosis that leads to arteriolar constriction reducing CBV and ICP. When the opposite occurs, that is, increased dissolved CO2 levels, it leads to a relative CSF acidosis accompanied by smooth muscle relaxation with increased CBF, CBV, and ICP. Since hypercapnia increases ICP, it is strictly avoided in neuroanesthesia.
5.
PaO2 reactivity: CBF reacts to a lesser extent to changes in PaO2 than to PaCO2. Hyperoxia is associated with minimal decreases in CBF (10–15 %), but hypoxemia (PaO2 < 60 mmHg) leads to a profound increase in CBF.
6.
Temperature: CBF changes by 5–7 % for every degree Celsius change in temperature. Hypothermia decreases CBF and cerebral metabolic rate, while hyperthermia has opposite effects.
Intracranial Pressure
The cerebrospinal fluid (CSF) is an ultrafiltrate of the plasma. CSF is produced at a rate of about 20 ml/h, or 500 ml/day, with a total volume of about 150 ml. It is a clear and colorless fluid produced in the brain by modified ependymal cells in the choroid plexus (about 50–70 %), while the remainder is formed around blood vessels and along ventricular walls. The CSF flows in a pulsatile manner and circulates as shown in Fig. 29.3.


Fig. 29.3
Circulation of cerebrospinal fluid
Normal CSF pressure is about 8–15 mmHg with the patient lying on the side and 16–24 mmHg with the patient sitting up. In newborns, CSF pressure ranges from 4.4 to 7.3 mmHg, about half that of adults. The CSF acts as a cushion or buffer for the brain and provides mechanical and immunological protection to the brain. It provides buoyancy to the brain by decreasing its effective mass from 1,400 to 47 g. The CSF also protects the brain tissue from injury and prevents brain ischemia. The CSF provides nutrients and clears metabolic waste from the central nervous system through the blood–brain barrier.
The cranial vault is a fixed space that provides protection but also represents a physical constraint that often results in increased ICP that reduces CPP. The vault contains the brain, blood, and CSF, with both the blood and CSF being incompressible. In the average adult, the skull encloses a total volume of about 1,500 ml (1,200 ml brain, 150 ml CSF, and 150 ml blood) (Fig. 29.4). The Monro–Kellie hypothesis states that the sum of the intracranial volumes of blood, brain, CSF, and other components is constant. To prevent an increase in ICP, any increase in the volume of one component must be compensated by a reciprocal decrease in the volume of another component. Sufficient buffering capacity (elastance) exists to allow for minor changes in the volume of any one component (tumor, CSF, extravascular blood, or edema) without significantly altering the ICP (Fig. 29.5).



Fig. 29.4
Contents of the cranial vault

Fig. 29.5
Intracranial elastance. As the intracranial volume increases, compensation mechanisms are exhausted and small changes in volume drastically increase the ICP
Initially the increased ICP shifts venous blood out of the skull; however, it is the extracranial shift of CSF that provides long-term adaptation to chronically increased ICP. An extraventricular drain (EVD) can be inserted by a neurosurgeon at the bedside to drain CSF through the ventricles, to both monitor and reduce the ICP. When these fluid shifts fail to control ICP, it increases and leads to reduced CPP. The brain’s response to reduced CPP is vasodilation to reestablish blood flow, but vasodilation increases CBV and ICP, which further worsens ischemia via a positive feedback loop (Fig. 29.6). Without emergent intervention, the compensatory mechanisms will be exhausted, and further ICP increase may force the brain out of an opening in the skull (herniation).


Fig. 29.6
The relationship of pathology, vasodilation, and neurophysiology on cerebral perfusion. A decrease in MAP or an increase in the volume of intracranial contents decreases perfusion, and the corresponding vasodilation worsens the pathologic state (MAP mean arterial pressure, CPP cerebral perfusion pressure, CBV cerebral blood volume, ICP intracranial pressure)
Common causes and clinical signs of increased ICP are summarized in Tables 29.1 and 29.2, respectively. Brainstem ischemia is a late sign of intracranial hypertension and raises the possibility of brain herniation and the associated demise. Because the brainstem regulates its own perfusion, brainstem ischemia triggers an increase in cardiac efferent activity, resulting in hypertension. Carotid baroreceptors sense the hypertension and transmit a signal to the brainstem via the glossopharyngeal nerve (CN IX) and initiate a parasympathetic response via the vagus nerve (CN X) that results in bradycardia. Hypertension and bradycardia in the presence of intracranial hypertension are known as Cushing’s phenomenon. This is actually a protective mechanism, and the hypertension itself should only be treated if the patient’s systolic pressure is excessive or accompanied by organ dysfunction.
Table 29.1
Causes of increased intracranial pressure
Intracranial | • Brain tumor • Cerebral edema, increased cerebral blood volume • Aneurysm rupture • Head injury • Hematoma (subdural, epidural) • Stroke • Hydrocephalus • Benign or idiopathic intracranial hypertension • Pneumocephalus • Abscess, cysts |
Extracranial | • Hypoventilation (hypercarbia, hypoxia) • Hypertension (pain, coughing) • Hypotension (hypovolemia) • Airway obstruction • Seizures • Hyperpyrexia • High altitude • Hepatic failure |
Table 29.2
Clinical signs of increased intracranial pressure
Early | • Headache • Vomiting • Mental status changes • Seizures • Pupil dysfunction • Cranial nerve dysfunction • Sunsetting sign |
Late | • Further deterioration in consciousness • Papilledema, pupillary dilation with decreased response to light • Bulging fontanels • Posturing, decreased spontaneous movements • Cushing’s triad (hypertension, bradycardia, irregular respiration) |
Strategies to Reduce ICP (Fig. 29.7)

Fig. 29.7
Physiologic, anesthetic, and surgical interventions to reduce intracranial pressure (MAP mean arterial pressure, CPP cerebral perfusion pressure, CBV cerebral blood volume, ICP intracranial pressure)
1.
Head elevation: Elevating the patient’s head increases jugular venous drainage and reduces cerebral venous pressure and volume, which in turn reduces ICP. This effect is maximized at a 30° angle, with further elevation decreasing the cerebral arterial pressure and reducing perfusion. Head elevation should be avoided in patients with hypovolemia, as it may further decrease blood pressure and reduce CPP.
2.
Hyperosmolar therapy: Increases blood osmolality so that water diffuses down its concentration gradient from the brain’s extracellular space into the bloodstream. The result is a decreased total brain volume and increased elastance. Mannitol or hypertonic saline (3–23 %) is often used for this purpose in patients with an intact blood–brain barrier.
Mannitol not only decreases brain volume but also decreases blood viscosity, which increases oxygen delivery to the brain. However, care should be taken when administering mannitol to cardiac-compromised patients, as it initially increases intravascular blood volume by drawing water into the bloodstream, which may cause congestive heart failure (CHF). Secondly, excessive diuresis with mannitol may cause hypovolemia. Maintaining euvolemia is of prime importance, and as such the maximum recommended serum osmolality is 320 mOsm/l. Mannitol is given in a dose of 0.25–1 g/kg and starts lowering ICP within 5 min, with a peak effect in 30–60 min and duration of action of 2–6 h.
Hypertonic saline is especially useful in hypovolemic and hypotensive patients. Adverse effects of administration include hypernatremia and hypervolemia, which may cause CHF, bleeding secondary to decreased platelet aggregation and prolonged coagulation times, hypokalemia, and hyperchloremic acidosis. Rapid administration of hypertonic saline, in the presence of hyponatremia, may increase the risk of central pontine myelinolysis.
3.
Hyperventilation: It can be used to temporarily reduce elevated ICP until medical therapy or a surgical intervention can remedy the underlying cause. In ICU patients with elevated ICP, hyperventilation has been used to produce hypocapnia for extended periods. However, existing data suggests that hypocapnea should only be used for brief periods of time, in order to either stop impending herniation or to alter total intracranial volume to provide surgical exposure.
Hypocapnea causes a change in CSF pH; however, the buffering mechanisms in the CSF return the pH to normal after 4–8 h. Over that time, both CBV and ICP slowly normalize, and further hypocapnea is required to reduce CBV. Without intervention, a return to eucapnea (PaCO2 ~ 40 mmHg) will cause CSF acidosis that will increase CBF and CBV. If an area of the brain was damaged, the resulting hyperemia may lead to a reperfusion injury with edema or intraparenchymal hemorrhage. Other changes that limit the therapeutic benefits of hyperventilation include:
(a)
Hypocapnea causes a leftward shift of the oxyhemoglobin dissociation curve and reduces oxygen delivery to tissues.
(b)
Vasoconstriction induced by hypocapnea reduces oxygen delivery to damaged ischemic tissue.
(c)
Hypocapnea has a limited impact on CBV and ICP. Of the 150 ml of CBV, only ~1/3rd of it (50 ml) is arterial with only a fraction of that blood in CO2-reactive arterioles. The result is that if hypocapnea is used to reduce CBF by 30 %, CBV is reduced only by ~7 %, which represents ~1 % of total intracranial volume at the expense of a dramatic reduction in perfusion.
4.
Steroid therapy: Steroids may be used (dexamethasone) to decrease brain swelling and for stabilizing the cell membrane in patients with increased ICP due to mass lesions, such as brain tumors and abscesses, inflammation, and infections. However, corticosteroids are not indicated in the management of increased ICP after traumatic brain injury.
5.
Seizures: They increase cerebral metabolic rate and the ICP and hence should be aggressively managed.
6.
Sedation and analgesia: Agitation and pain increase ICP. Therefore, providing anxiolysis (midazolam, propofol) and adequate analgesia (morphine) is an important aspect in the management of ICP.
7.
Adequate ventilation: Optimal respiratory management to prevent hypoxia and hypercarbia is extremely important. A ventilated patient may require neuromuscular blockade for effective ventilator use and prevention of raised ICP. It should be noted that excessive PEEP can increase ICP by decreasing venous return and increasing cerebral venous pressure.
8.
Barbiturate coma: Refractory cases of increased ICP may require inducing a barbiturate coma and hypothermia. However, a rebound increase in ICP may occur when discontinuing these therapies.
9.
Surgical interventions: To reduce intracranial pressure include removing an intracranial mass or hemorrhage, decompressive craniectomy, or draining excess CSF via a ventriculostomy catheter. Resecting portions of the cranium and underlying dura nullifies the Monro–Kellie doctrine such that the brain is no longer confined to the skull.
Effects of Anesthetics on Cerebral Physiology
All anesthetic agents impact CMRO2, cerebral arteriolar tone, and the brain’s reactivity to changes in carbon dioxide tension, with resultant changes in CBF, CBV, and ICP (Table 29.3).
Table 29.3
Effects of common anesthetic agents on neurophysiology
Anesthetic agent | CBF | CMRO2 | ICP | Comments | |
---|---|---|---|---|---|
Thiopental | ↓ | ↓↓ | ↓ | No longer available in the USA | |
Etomidate | ↓ | ↓ | ↓ | Adrenal suppression is a concern | |
Propofol | ↓ | ↓ | ↓ | May decrease MAP profoundly | |
Ketamine | ↑↑ | ↑ | ↑↑ | Undesirable neuroanesthetic | |
Midazolam | ↓ | ↓ | ↓ | Variable reaction to small doses | |
Fentanyl | ↓ | – | ↓ | Long-duration infusions may cause hypercarbia | |
Remifentanil | ↓ | – | ↓ | Half-life ~3–5 min, effective for periods of intense stimulation | |
Nitrous oxide | ↑↑ | ↑ | ↑ | Controversial neuroanesthetic | |
Desflurane, isoflurane, sevoflurane | <0.6 MAC | – | ↓ | – | PaCO2 reactivity preserved |
>0.6 MAC | ↑ | ↓↓ | ↑ | Vasodilation and increased CBF and ICP | |
Halothane | ↑↑ | ↓ | ↑ | Extreme cerebral vasodilation |
Intravenous Anesthetics
Thiopental, propofol, and etomidate reduce CMRO2 and CBF in tandem. Barbiturates produce vasoconstriction, which reduces CBF and CMRO2 in normal brain tissue. Blood flow is redistributed to ischemic areas, which remain dilated (reverse steal phenomenon). The maximal reduction in CBF and CMRO2 is about 50–60 %, after which the EEG becomes isoelectric. All these changes lead to a decrease in the ICP. When the cranium has been opened during an intracranial procedure, CBF reduction produced by these agents improves surgical exposure. In addition to reducing the ICP, barbiturates have anticonvulsant properties. However, methohexital, which is commonly used for inducing patients for electroconvulsive therapy (ECT), activates seizure foci when used in smaller doses. Higher doses of methohexital have similar anticonvulsant properties as thiopental.
Propofol has similar actions to thiopental: producing reductions in ICP and having anticonvulsant properties. The advantage of using propofol is its short duration of action: however, it produces more hypotension than thiopental, which can significantly reduce cerebral perfusion pressure, especially in cardiac-compromised patients. Etomidate, like thiopental and propofol, reduces both the CBF and ICP. However, etomidate produces adrenal suppression and is associated with myoclonic movements on administration.
The effects of ketamine are different than other intravenous induction agents. Ketamine increases CBF (cerebral vasodilation), which leads to an increase in ICP. Ketamine increases activity in the limbic system and decreases activity in other brain areas, so-called dissociative anesthesia.
Opioids minimally impact CBF and CMRO2. Fentanyl is usually preferred over morphine in neuroanesthesia, because of the latter’s slow onset and prolonged duration of action. Excessive administration of opioids, however, may cause respiratory depression, which may increase the PaCO2 and the ICP. Benzodiazepines lower CBF and CMRO2; however, their effects are milder compared to barbiturates. Benzodiazepines are usually agents of first choice for treating seizures. The duration of action of benzodiazepines is longer compared to other intravenous induction agents.
Muscle relaxants do not directly affect brain physiology. However, some muscle relaxants cause a release of histamine, which causes cerebral vasodilation and an increase in CBF and ICP. Succinylcholine increases muscle spindle activity, which transiently increases the ICP. This effect is usually blunted by prior administration of the intravenous induction agent or a small defasciculating dose of a nondepolarizing muscle relaxant.
Inhalational Anesthetics
All volatile anesthetics depress CMRO2 with increasing doses. Volatile anesthetics also produce cerebral vasodilation with resultant increase in CBF. However, at concentrations <0.6 MAC, the vasodilatory effect is minimal, and anesthesiologists commonly utilize volatile anesthetics for their easy titrability and fast, predictable elimination. Concentrations >0.6 MAC are avoided because they lead to dose-dependent cerebral vasodilation that increases CBF, CBV, and ICP. This is commonly referred to as uncoupling of CMRO2 and CBF. Volatile anesthetics increase blood flow to normal areas of the brain; therefore, ischemic brain areas see a decline in their perfusion (steal phenomenon). Among volatile anesthetics, halothane increases CBF the greatest and decreases the CMRO2 the least.
Nitrous oxide (N2O) has been shown to mildly increase CMRO2 and CBF. When combined with a volatile anesthetic, these effects are additive. N2O is also known to rapidly diffuse into closed spaces that may cause a rapidly expanding venous air embolism or a pneumocephalus. However, it has been found that the use of N2O does not significantly change outcomes when used during craniotomies. Some anesthesiologists still use N2O for its rapid titrability and analgesic properties.
Anesthesia for neurosurgical patients is often a combination of a low-dose volatile anesthetic (<0.6 MAC) and an intravenous anesthetic infusion to provide favorable CBV. Since the residual effects of anesthetics can hinder the postoperative evaluation of neurosurgical patients, a combination of fast-offset agents is often preferred. One common combination is 0.6 MAC of sevoflurane/desflurane, combined with propofol and remifentanil infusions.
Vasoactive Drugs
Catecholamine infusions are commonly used in patients to maintain MAP and CPP. When the CPP is within the range of autoregulation and the blood–brain barrier is intact, catecholamine infusions do not impact CBF. However, in pathologic areas, CBF may be a linear function of CPP, and slight changes in CPP could dramatically impact tissue perfusion. Phenylephrine and norepinephrine are common choices of vasopressors for neuroanesthesia.
When the blood–brain barrier (BBB) is compromised, epinephrine can interact directly with pathologic tissues and increase CMRO2. Because dopamine is involved in the hypothalamic–pituitary axis, its infusions are typically avoided. Direct cerebral vasodilators such as hydralazine and nitroprusside have a greater impact on CBF and ICP. Oral nimodipine and parenteral nicardipine are cerebral vasodilators that are hypothesized to reduce cerebral vasospasm in blood vessels serving pathologic tissue.
Cerebral Protection
Pathophysiology of Cerebral Ischemia
The mechanism of the cerebral ischemia is important to the pathogenesis of the disease. For example, cardiac arrest causes global ischemia without collateral flow, causing neuronal depolarization within minutes. In subarachnoid hemorrhage, there is focal ischemia as well as an adjacent area that receives some, albeit reduced, collateral perfusion. The ischemic area is known as the core and the adjacent area the penumbra. The imbalance between perfusion and CMRO2 in the penumbra must be restored in a timely manner so that it can be saved.
No matter the cause, ischemic cells release toxic products and the excitatory amino acid glutamate that increase energy consumption at the border between the ischemic core and the penumbra, leading to depolarization in adjacent cells. In a futile attempt to repolarize, these cells consume their energy stores leading to expansion of the ischemic core and further injury (Fig. 29.8). This mechanism of injury is known as cortical spreading depression and commonly occurs in ischemic tissue after subarachnoid hemorrhage (SAH) and traumatic brain injury (TBI).


Fig. 29.8
Pathophysiology of cerebral ischemia
A dramatic change in the balance between oxygen metabolic supply and demand, such as a regional decrease in CPP or a seizure, can trigger a large group of at-risk cells in the penumbra to depolarize. The depolarization of an already injured cell can be massive with large influxes of Na+ and Ca++. The energy required to remove these ions can double the CMRO2. If this metabolic requirement is not met, K+ and organic anions (notably glutamate) flow out of the cell where they enhance depolarization of local cells by reducing the K+ potential and opening excitatory channels in surrounding cells. This mechanism spreads the depression. Hemorrhaged blood increases extracellular K+, which increases the energy required for repolarization. Free hemoglobin also reduces nitric oxide (NO), inhibiting vasodilation and activating apoptotic pathways, leading to increased risk of ischemia. Water diffuses down the sodium and calcium gradients, which leads to edema and further pathology.
If the CBF–CMRO2 coupling mechanism is still intact, the spreading depolarization is counteracted with spreading hyperemia. Hyperemia lasts for only a few minutes, which is just enough time for perfusion to match the increased metabolic demand so that the neurons can recover (repolarize). Hyperemia is followed by a period of oligemia with failures of CBF–CMRO2 coupling and PaCO2 autoreactivity that lasts for a few hours.
Strategies for Cerebral Protection
Cerebral protection (CP) is a term that refers to strategies to prevent long-term deterioration of CNS function after some insult. That insult may be planned, such as surgery, or impromptu as in cardiac arrest, a cerebrovascular accident (CVA), traumatic brain injury (TBI), or spinal cord injury. These conditions occur frequently, with 750,000 CVAs and 1.7 million TBIs per year in the USA alone. Several strategies have been evaluated for cerebral protection, with the hope that instituting therapies prior to the insult (prevention) may increase their efficacy.
For decades physicians have been exploring means to protect neural tissue from ischemic injury. They initially focused on limiting oxygen demand (decreasing CMRO2), so that the reduced oxygen supply as a result of ischemia could match the CMRO2. Maximal CMRO2 reduction that can be achieved is ~60 %, which coincides with cessation of electrical activity and burst suppression on EEG. Animal study protocols that dosed volatile and intravenous agents to achieve burst suppression protected neural tissue from ischemic injury of brief to moderate duration. However, the tissue died several days later.
When these experiments were repeated and antiapoptotic drugs were administered a few days after the insult, the cells survived. Further experiments determined that only 1/3 of the barbiturate dose that is required to achieve an isoelectric EEG provided near-maximal protection. Therefore, neuroprotection is accomplished by a different, as yet undefined, mechanism that may include reductions in the stress response, slowing the apoptotic cascade, or encouraging axonal regeneration. Several triggers and pathways of apoptosis have been elucidated, including calcium dysregulation, free radical damage, and cytokine release by CNS macrophages (microglial cells). All activate enzymes that cause apoptosis. Multiple laboratory studies have shown that by blocking a single pathway, apoptosis can be delayed.
Anesthetic agents have been shown to provide varying degrees of neuroprotection either by reducing the metabolic demand and the stress response or by blocking steps in the apoptosis cascade. Anesthetics reduce metabolic demand by hyperpolarization of mitochondrial potassium ion channels; reduce excitation secondary to GABA agonism, NMDA antagonism, and reduced glutamate release; and reduce CMRO2 that both saves energy for cellular maintenance and limits free radical formation and membrane oxidation. Strategies for cerebral protection are summarized in Table 29.4.
Table 29.4
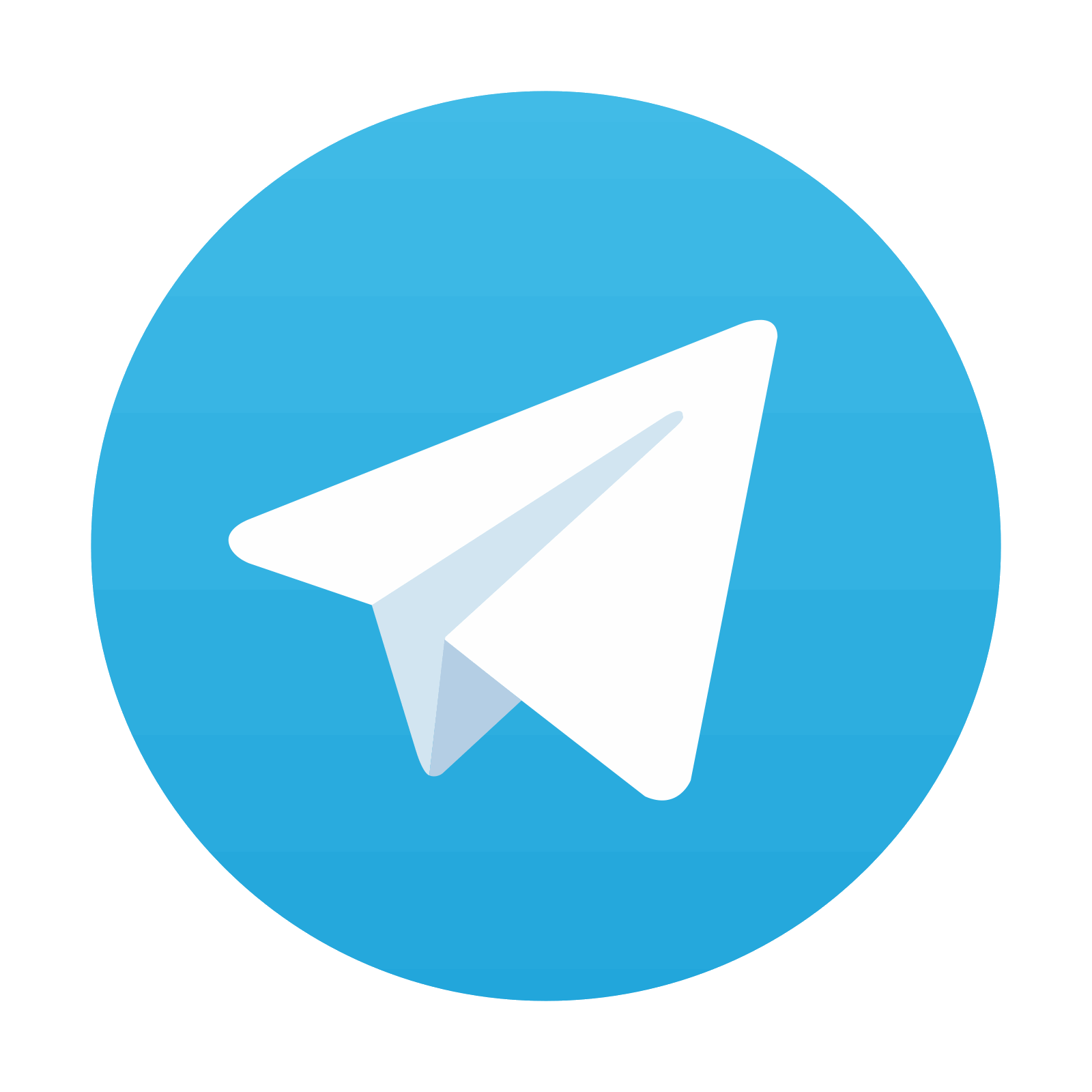
Strategies for cerebral protection
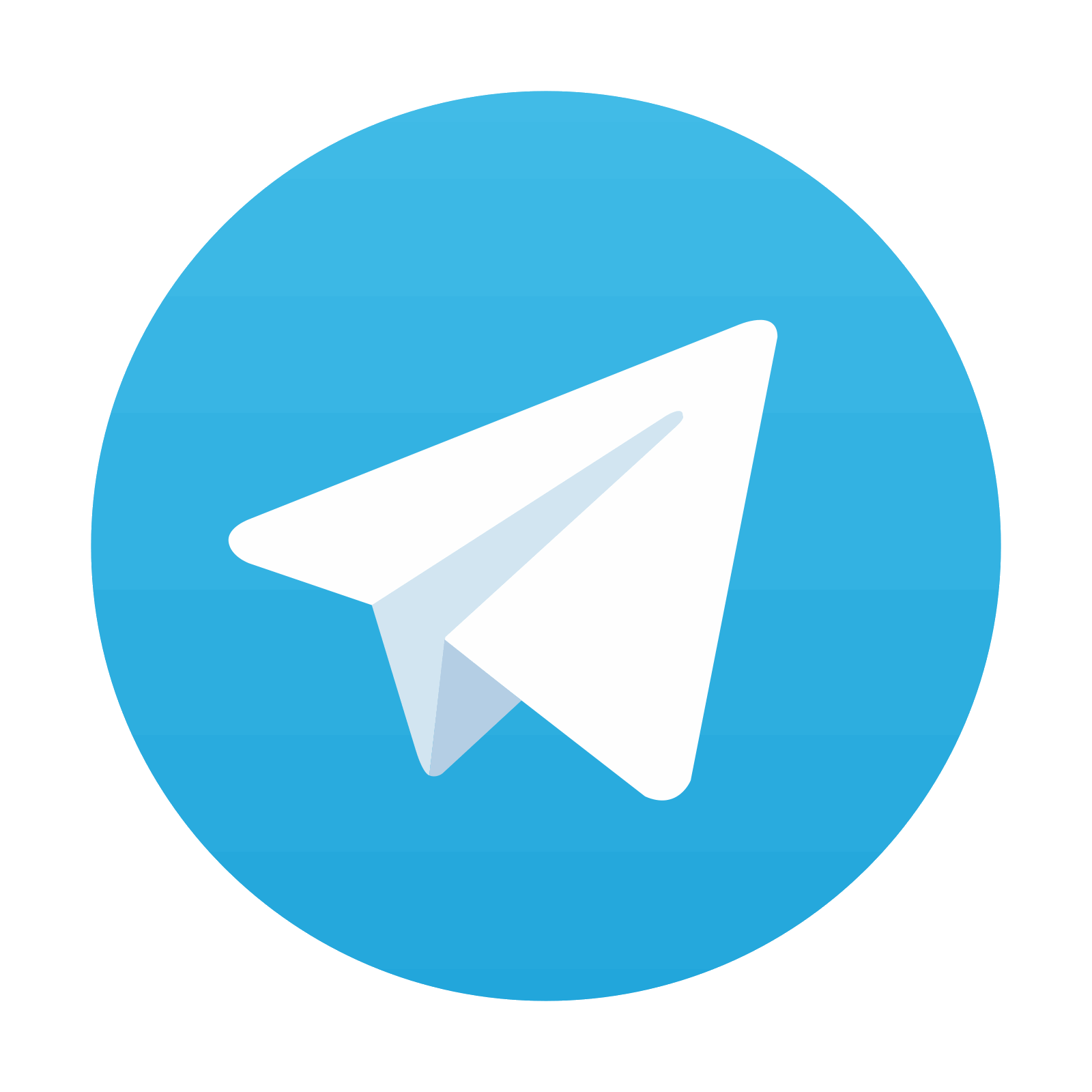
Stay updated, free articles. Join our Telegram channel
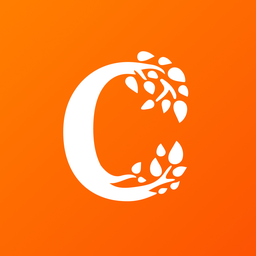
Full access? Get Clinical Tree
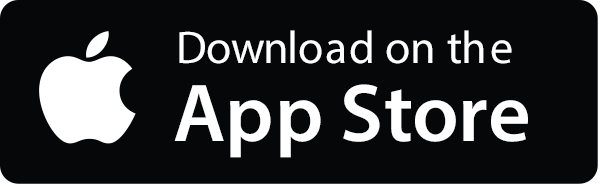
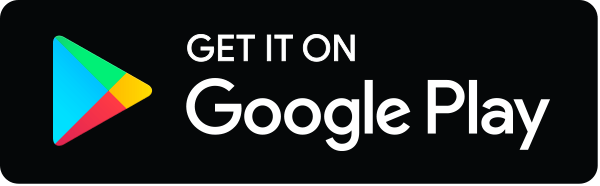
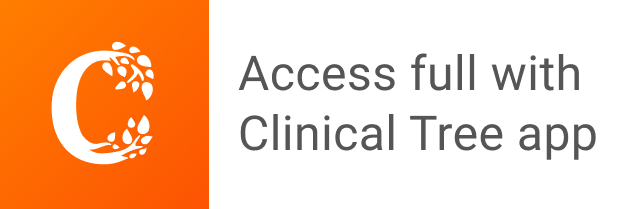