Fig. 18.1
Pain pathways. Illustration of the postsynaptic dorsal column (PSDC) and the spinothalamic tract (STT) as they arise in the dorsal horn of the spinal cord and ascend towards the brainstem and eventually the thalamus. The PSDC pathway synapses on neurons in the dorsal column nuclei (DCN; the nucleus gracilis is labeled); axons of DCN neurons subsequently cross the midline and ascend in the medial lemniscus to converge onto thalamic neurons. The axons of STT neurons cross the midline at the dorsal horn level and ascend to the VPL nucleus of the thalamus in the anterolateral quadrant of the cord. Peripheral input is shown from the colon mainly onto the PSDC pathway and from the skin mainly onto the STT to illustrate the relative importance of these pathways in the nociception arising from these tissues respectively
Representation of Nociceptive Sensation in the Brain
In contrast to most other sensory modalities, the neuroanatomical substrates in the brain for pain sensation in general and visceral pain in particular, have only recently begun to be elucidated. Major advances in this field have come through functional anatomical and physiological studies in nonhuman primates and rats, which have identified substrates that underlie findings from functional imaging and microelectrode studies in humans.
Thalamic Representation of Pain
Electrophysiological recordings made of nociceptive responses in the VPL and ventral posteromedial (VPM) nuclei of the thalamus in rats and monkeys by many investigators showed that neurons in these thalamic nuclei can be activated by nociceptive stimulation of the periphery (Al-Chaer et al. 1996a; Apkarian and Shi 1994; Brüggemann et al. 1994; Bushnell and Duncan 1987; Bushnell et al. 1993; Casey and Morrow 1983, 1987; Chandler et al. 1992; Chung et al. 1986; Duncan et al. 1993; Gaze and Gordon 1954; Kenshalo et al. 1980; Pollin and Albe-Fessard 1979; Yokota et al. 1988).
In general, responses of nociceptive neurons in the VPL nucleus to innocuous cutaneous mechanical stimuli are weak, in contrast to their responses to noxious mechanical stimuli (Casey and Morrow 1983, 1987; Chung et al. 1986; Kenshalo et al. 1980). The location of the neurons in the VPL nucleus is somatotopic but their receptive fields are relatively small and situated on the contralateral side. Almost all of the VPL neurons tested were shown by antidromic activation to project to the primary somatosensory cortex (SI) (Kenshalo et al. 1980). Surprisingly, most neurons (85%) in the VPL nucleus respond to both cutaneous and visceral stimuli (Al-Chaer et al. 1996a, 1998b; Brüggemann et al. 1994; Chandler et al. 1992). Although the cutaneous input is somatotopic, the visceral input is not viscerotopic (Brüggemann et al. 1994).
Investigations of visceral inputs into the thalamus were made using electrical stimulation of visceral nerves (Aidar et al. 1952; Dell and Olson 1951; McLeod 1958; Patton and Amassian 1951) or natural stimulation of visceral organs (Chandler et al. 1992; Davis and Dostrovsky 1988; Emmers 1966; Rogers et al. 1979). In monkeys, the medial thalamus receives viscerosomatic input via thoracic STT neurons (Ammons et al. 1985), whereas neurons in the lateral thalamus are activated by input through the STT and the DC (Al-Chaer et al. 1998a). Lateral thalamic neurons can also be excited by colorectal distension or urinary bladder distension and by convergent input elicited by noxious stimulation of somatic receptive fields in proximal lower body regions (Chandler et al. 1992). In fact, the majority of lateral thalamic somatosensory neurons in squirrel monkeys receive somatovisceral and viscero-visceral inputs from naturally stimulated visceral organs (Brüggemann et al. 1994). In the rat, neurons in and near the thalamic ventrobasal complex respond to stimulation of different visceral organs, including the uterus, the cervix, the vagina, and the colon (Al-Chaer et al. 1996a; Berkley et al. 1993). Colorectal distension or colon inflammation excites neurons in the ventral posterolateral nucleus of thalamus (Al-Chaer et al. 1996a; Berkley et al. 1993; Brüggemann et al. 1994) and in the medial thalamus at the level of the nucleus submedius (Kawakita et al. 1997).
Microstimulation in the region of the thalamic principal sensory nucleus (the ventrocaudal nucleus) – a nucleus that corresponds to the ventral posterior nucleus in the cat and the monkey (Hirai and Jones 1989; Jones 1985) – can evoke a sensation of angina in humans (Lenz et al. 1994) and trigger in some cases pain “memories” (Davis et al. 1995). Electrical stimulation of the thalamic ventrobasal complex in animals inhibits viscerosensory processing in normal rats but facilitates visceral hypersensitivity in rats with neonatal colon pain (Saab et al. 2004). These observations coupled with an extensive repertoire of experimental data suggest that the thalamus, particularly the posterolateral nucleus, is involved in the processing of visceral information, including both noxious and innocuous visceral inputs.
Nociceptive neurons also exist in the VPI and POm nuclei (Casey and Morrow 1987; Apkarian and Shi 1994; Pollin and Albe-Fessard 1979). The cutaneous receptive fields of neurons in the VPI nucleus are somatotopically organized but tend to be larger than those of the VPL nociceptive neurons and presumably project to the secondary somatosensory cortex (SII) (Friedman et al. 1986). The cells studied in the monkey POm nucleus had small, contralateral nociceptive receptive fields. The POm nucleus projects to the retroinsular cortex in monkeys (Burton and Jones 1976).
Cortical Pain Processing
Anatomical, physiological, and lesion data implicate multiple cortical regions in the complex experience of pain (Kenshalo et al. 1988; White and Sweet 1969). These regions include primary and secondary somatosensory cortices, anterior cingulate cortex, insular cortex, and regions of the frontal cortex. Nevertheless, the role of different cortical areas in pain processing remains controversial. Studies of cortical lesions and cortical stimulation in humans did not uncover a clear role of various cortical areas in the pain experience and more recent human brain-imaging studies are not always consistent in revealing pain-related activation of somatosensory areas (see Bushnell et al. 1999). Despite this controversy, the application of functional magnetic resonance imaging (fMRI) and positron emission tomography (PET) has identified a network of brain areas that process painful sensation from a number of somatic regions including chronic pain states (see Apkarian et al. 2005; Matre and Tran 2009; Peyron et al. 2000; Veldhuijzen et al. 2007) and from a number of visceral organs such as the esophagus (Aziz et al. 1997), stomach (Ladabaum et al. 2001), and the anorectum (Hobday et al. 2001). Results of these studies show activation of the primary somatosensory cortex (SI) by a range of noxious stimuli. These studies also confirm the somatotopic organization of SI pain responses, thus supporting the role of SI in pain localization. Other imaging data that implicate SI in the sensory aspect of pain perception note that S1 activation is modulated by cognitive manipulations that alter perceived pain intensity but not by manipulations that alter unpleasantness, independent of pain intensity (Baliki et al. 2006).
Visceral sensation, on the other hand, is primarily represented in the secondary somatosensory cortex (SII). Unlike somatic sensation, which has a strong homuncular representation in SI, visceral representations in the primary somatosensory cortex are vague and diffuse (Aziz et al. 1997). This might account for visceral sensation being poorly localized in comparison with somatic sensation. Nevertheless, visceral sensation is represented in paralimbic and limbic structures (e.g., anterior insular cortex, amygdala, anterior and posterior cingulate cortex), and prefrontal and orbitofrontal cortices (Mertz et al. 2000; Silverman et al. 1997), areas that purportedly process the affective and cognitive components of visceral sensation (Derbyshire 2003).
Differential cortical activation is also seen when comparing sensation from the visceral and somatic regions of the gastrointestinal tract, for example, sensations from the esophagus vs. the anterior chest wall (Strigo et al. 2003) or the rectum vs. the anal canal (Hobday et al. 2001). Brain processing for esophageal and anterior chest wall sensations occurs in a common brain network consisting of secondary somatosensory and parietal cortices, thalamus, basal ganglia, and cerebellum (Strigo et al. 2003). Yet, differential processing of sensory information from these two areas occurred within the insular, primary sensory, motor, anterior cingulate, and prefrontal cortices. These findings are consistent with other studies which highlight similarities in the visceral and somatic pain experience and might also explain the individual’s ability to distinguish between the two modalities and generate differential emotional, autonomic, and motor responses when each modality is individually stimulated.
Descending Modulatory Pathways
In addition to the afferent pathways that process nociceptive signals at different levels of the neuraxis, pain processing involves a number of modulatory controls that exist throughout the nervous system and function to enhance or dampen the intensity of the original signal or to modify its quality. At the level of the spinal cord input from non-nociceptive and nociceptive afferent pathways can interact to modulate transmission of nociceptive information to higher brain centers. In addition, the brain contains modulatory systems that affect the conscious perception of sensory stimuli. Spinal nociceptive transmission is subject to descending modulatory influences from supraspinal structures e.g., periaqueductal gray, nucleus raphe magnus, locus coeruleus, nuclei reticularis gigantocellularis, and the ventrobasal complex of the thalamus (see Besson and Chaouch 1987; Hodge et al. 1986; Light 1992; Peng et al. 1996b; Willis 1982). Descending modulation can be inhibitory, facilitatory or both depending on the context of the stimulus or the intensity of the descending signal (Saab et al. 2004; Zhuo and Gebhart 2002). The descending influence from the ventromedial medulla is mediated mainly by pathways traveling in the dorsolateral spinal cord (Zhuo and Gebhart 2002) and can be inhibitory or facilitatory based on stimulus intensity. In contrast, descending control from the thalamus is context-specific in that it may facilitate or inhibit spinal nociceptive processing depending upon the presence or absence of central sensitization (Saab et al. 2004). For instance, serotonergic (Peng et al. 1995), noradrenergic (Peng et al. 1996a; Proudfit 1992), and to a lesser extent dopaminergic projections are major components of descending modulatory pathways (Dahlström and Fuxe 1964, 1965), in addition to a major role played by opiates and enkephalins (Duggan and North 1984; Duggan et al. 1977).
Molecular Pathways
The activity of nociceptors can be affected by adequate stimuli, such as strong mechanical, thermal, or chemical stimuli (see Willis 1985; Willis and Coggeshall 2004), and also by chemical actions on surface membrane receptors of their axons. A battery of chemical mediators, including biogenic amines (such as glutamate, [gamma]-aminobutyric acid (GABA), histamine, serotonin, norepinephrine) (Dray et al. 1994; McRoberts et al. 2001), opiates (Joshi et al. 2000; Su et al. 1997), purines, prostanoids, proteases, cytokines, and other peptides (such as bradykinin, substance P, and CGRP) act in a promiscuous manner on a range of receptors expressed upon any one sensory ending (Kirkup et al. 2001).
Three distinct processes are involved in the actions of these substances on afferent nerves. First, by direct activation of receptors coupled to the opening of ion channels present on nerve terminals, the terminals are depolarized and firing of impulses is initiated. Second, by sensitization that develops in the absence of direct stimulation and results in hyperexcitability to both chemical and mechanical modalities, e.g., opiate receptors are ineffective in modulating the normal activity of joint nociceptors, but they were shown to become effective after the development of inflammation (Stein 1994). Sensitization may also involve postreceptor signal transduction that includes G-protein coupled alterations in second-messenger systems which in turn lead to phosphorylation of membrane receptors and ion channels that control excitability of the afferent endings. Third, by genetic changes in the phenotype of mediators, channels, and receptors expressed by the afferent nerve, for example after peripheral nerve injury, many afferent fibers express newly formed adrenoreceptors (Bossut and Perl 1995; Campbell et al. 1992; Sato and Perl 1991; Xie et al. 1995). A change in the ligand-binding characteristics or coupling efficiency of these newly expressed receptors could alter the sensitivity of the afferent terminals. Neurotrophins, in particular nerve growth factor and glial-derived neurotropic factor, influence different populations of visceral afferents and play an important role in adaptive responses to nerve injury and inflammation (McMahon 2004; Bielefeldt et al. 2006).
Ion channels on nociceptors act as molecular transducers that depolarize these neurons, thereby setting off nociceptive impulses along the pain pathways (Price 2000; Costigan and Woolf 2000). Among these ion channels are the members of the transient receptor potential (TRP) family, activated by changes in temperature and extracellular pH and the presence of vanilloid ligands, such as capsaicin and mustard oil (Woolf and Ma 2007). The thermosensitive TRP family constitutes an interface with the environment and includes a number of diverse channels, each with a distinct thermal threshold and a different set of chemical activators. To date, the most studied member of the TRP family is the TRPV1 receptor (Di Marzo et al. 2002; Cortright and Szallasi 2004). Activation of primary afferents can also be brought about by other types of ion channels, including the degenerin epithelial sodium channel (ENaC) family (Kellenberger and Schild 2002; Price et al. 2001) and the acid-sensing ion channel (ASIC) family, of which ASIC3 has been implicated in the perception of noxious mechanical stimulation in mammals (Mogil et al. 2005). Tissue damage also results in the release of high concentrations of ATP, which can act on ligand-gated purinergic P2X channels and P2Y receptors to activate nociceptors (Khakh and North 2006).
Voltage-gated sodium channels (VGSCs) are also critical for the initiation of action potentials in the peripheral terminals of nociceptors and conduction along axons in peripheral nerves to the spinal cord. VGSCs allow sodium ion influx in response to local membrane depolarization, such as the potential generated in the peripheral terminals of nociceptors (Cummins et al. 2007). Different roles have been attributed to each sodium channel in influencing neuronal excitability, often associated with expression in specific subclasses of DRG neurons.
In the CNS, the central axon terminals of primary afferent neurons release neurotransmitters in the dorsal horn of the spinal cord. Like most neurons in the CNS, Aδ and C fiber nociceptors use glutamate as their fast neurotransmitter. Glutamate binds to ionotropic α-amino-3-hydroxy-5-methyl-4-isoxazolepropionic acid (AMPA) and N-methyl-d-aspartic acid (NMDA) receptors, as well as to metabotropic glutamate receptors expressed in the dorsal horn. AMPA receptors produce fast excitatory postsynaptic potentials that signal the onset, intensity, duration, and location of peripheral noxious stimulation. More-intense or sustained activation of C fiber nociceptors also results in the release of neuropeptide neuromodulators, such as substance P and the calcitonin-gene-related peptide (CGRP), which produce slow synaptic potentials via the neurokinin 1 receptor (NK1R) and the CGRP-1 and CGRP-2 receptors, respectively. The presence of these peptides enables considerable use-dependent functional plasticity in the control of pain transmission, since their release typically follows high-intensity stimulation (Schaible 2004; for a review, see Bingham et al. 2009).
Presynaptic voltage-gated calcium channels, such as CaV2.2, contribute substantially to controlling neurotransmitter release from synaptic vesicles in the dorsal horn. Neurotransmitter release is also regulated by presynaptic inhibition produced by the neurotransmitter γ-aminobutyric acid (GABA) acting on GABAA ion channels and GABAB receptors. Other presynaptic inhibitory receptors include the cannabinoid receptor 1 (CB1) and the three opioid receptors μ, δ, and κ. GABA also induces postsynaptic inhibition in dorsal horn neurons comparable to that caused by the neurotransmitter glycine (Woolf and Salter 2000). Each of the elements involved in the regulation of spinal neurotransmitter release constitutes potential targets for pain pharmacotherapy.
Multiple molecular mechanisms have been associated with producing the sensation of pain many of which have been largely developed through the use of genetic manipulations. These signaling pathways involve many molecular components that could potentially be targets for pharmacotherapeutic intervention, but the complexity of these systems might also mean that multiple sites must be affected simultaneously to disrupt propagation of pain signals or restore homeostatic conditions. This makes the idea of a “silver bullet” for the treatment of pain all the more challenging.
Gender Differences in Pain Processing
Gender differences are a hallmark of pain perception, particularly visceral pain; however, little is known about the biological causes of these differences (Fillingim 2000; Keogh 2009, see also Keogh 2011). Recent studies have shown that estrogen receptors located on nociceptive neurons in the spinal cord play an important role in the sexually differentiated responses of these neurons to nociceptive visceral stimuli (Al-Chaer, unpublished observations). Similarly gender differences have been reported in the cortical representation of pain. Activation in the sensory–motor and parieto-occipital areas is common in both males and females following rectal distension; however, greater activation in the anterior cingulate/prefrontal cortices has been found in women (Kern et al. 2001). These actual gender differences in the processing of sensory input substantiate reports that perceptual responses are exaggerated in female patients with chronic pain.
Conclusion
It is important to keep in mind that these pathways, while seemingly anatomically segregated and traditionally perceived as conveying specific perceptions of pain, are in fact functionally dynamic, interactive polymodal channels for visceral, cutaneous, muscular and proprioceptive sensations, in addition to possible motor, autonomic and not as yet defined functions. As such, the STT as well as the DC can be regarded as interactive polymodal channels for visceral, somatic, and autonomic events with sorted priorities for the sake of immediate, reliable, and simple readings of acute and transient but complex situations. These breakthroughs in defining pain mechanisms and pathways have advanced the field of pain research and management particularly in the areas of drug development. However, despite these extraordinarily impressive scientific advances in our understanding of the mechanisms of pain and describing some of its pathways, the field is beset by similarly and equally impressive stalemates and retreats in the actual management and cure of pain. After all, knowledge about pain and its mechanisms is only useful to the extent it helps the sufferer.
For pain relief, we naturally use anything that works; historically we used trephination, opiates, and willow bark. Today, regardless of their site of action, we continue to use some of the same techniques that worked albeit in different pharmaceutical formulae and more controlled environments… but the nervous system seems to be extremely resistant to switching off pain!
Future Directions
Translational research in the immediate and extended future can be expected to maintain ongoing progress in each of the following areas:
1.
Continued mechanistic focus on the basic science of pain that includes the molecular basis for peripheral sensitization of sensory receptors by inflammatory mediators, selectivity of central pain-related transmission pathways, and higher-order central processing of nociceptive information from the periphery.
2.
Integration of imaging technology and classic neurophysiological and neuropharmacological approaches for improved understanding of the neurobiology of pain.
3.
Expanded investigation of the psychoneuroendocrine pathways, which are not only responsible for alteration of function during psychogenic stress and the exacerbation of chronic pain states, but which also may be partly responsible for gender differences in pain.
4.
Enhanced focus on the identification of drug targets on neuronal elements of the nervous system and on non-neuronal cell types, such as glia, which release substances that alter the activity of neurons.
5.
More attention to individual differences in pain reporting and efficacy of management in addition to novel phenotype-directed approaches to pain study and treatment.
However, given the immediate need for pain relief in severe conditions refractory to conventional treatment and the reported success, albeit anecdotal, of many alternative and complementary therapies, focused research on the mechanisms of these therapies is likely to yield beneficial results in the immediate future.
Acknowledgments
The author would like to thank Ms. Kirsten Garner for assistance with editing the manuscript. This work was supported by NIH Grants DK077733, DK081628.
References
Aidar, O., Geohegan, W. A., & Ungewitter, L. H. (1952). Splanchnic afferent pathways in the central nervous system. Journal of Neurophysiology, 15, 131–138.PubMed
Albe-Fessard, D., Berkley, K. J., Kruger, L., Ralston, H. J., & Willis, W. D. (1985). Diencephalic mechanisms of pain sensation. Brain Research Reviews, 9, 217–296.
Albe-Fessard, D., Levante, A., & Lamour, Y. (1974a). Origin of spinothalamic and spinoreticular pathways in cats and monkeys. Advances in Neurology, 4, 157–166.
Albe-Fessard, D., Levante, A., & Lamour, Y. (1974b). Origin of spino-thalamic tract in monkeys. Brain Research, 65, 503–509.PubMed
Al-Chaer, E. D., Feng, Y., & Willis, W. D. (1998a). A role for the dorsal column in nociceptive visceral input into the thalamus of primates. Journal of Neurophysiology, 79, 3143–3150.PubMed
Al-Chaer, E. D., Feng, Y., & Willis, W. D. (1998b). Visceral pain: A disturbance in the sensorimotor continuum? Pain Forum, 7(3), 117–125.
Al-Chaer, E. D., Feng, Y., & Willis, W. D. (1999). A comparative study of viscerosomatic input onto postsynaptic dorsal column and spinothalamic tract neurons in the primate. Journal of Neurophysiology, 82(4), 1876–1882.PubMed
Al-Chaer, E. D., Kawasaki, M., & Pasricha, P. J. (2000). A new model of chronic visceral hypersensitivity in adult rats induced by colon irritation during postnatal development. Gastroenterology, 119(5), 1276–1285.PubMed
Al-Chaer, E. D., Lawand, N. B., Westlund, K. N., & Willis, W. D. (1996a). Visceral nociceptive input into the ventral posterolateral nucleus of the thalamus: A new function for the dorsal column pathway. Journal of Neurophysiology, 76, 2661–2674.PubMed
Al-Chaer, E. D., Lawand, N. B., Westlund, K. N., & Willis, W. D. (1996b). Pelvic visceral input into the nucleus gracilis is largely mediated by the postsynaptic dorsal column pathway. Journal of Neurophysiology, 76, 2675–2690.PubMed
Al-Chaer, E. D., & Traub, R. J. (2002). Biological basis of visceral pain: Recent developments. Pain, 96(3), 221–225.PubMed
Al-Chaer, E. D., & Willis, W. D. (2007). Neuroanatomy of visceral pain: Pathways and processes. In P. J. Pasricha, W. D. Willis, & G. F. Gebhart (Eds.), Chronic abdominal and visceral pain: Theory and practice. New York: Informa Health Care Inc.
Amassian, V. E. (1951). Fiber groups and spinal pathways of cortically represented visceral afferents. Journal of Neurophysiology, 14, 445–460.PubMed
Ammons, W. S. (1989a). Primate spinothalamic cell responses to ureteral occlusion. Brain Research, 496, 124–130.PubMed
Ammons, W. S. (1989b). Electrophysiological characteristics of primate spinothalamic neurons with renal and somatic inputs. Journal of Neurophysiology, 60, 1121–1130.
Ammons, W. S., Girardot, M. N., & Foreman, R. D. (1985). T2-T5 spinothalamic neurons projecting to medial thalamus with viscerosomatic input. Journal of Neurophysiology, 54, 73–89.PubMed
Angaut-Petit, D. (1975a). The dorsal column system: I. Existence of long ascending postsynaptic fibres in the cat’s fasciculus gracilis. Experimental Brain Research, 22, 457–470.
Angaut-Petit, D. (1975b). The dorsal column system: II. Functional properties and bulbar relay of the postsynaptic fibres of the cat’s fasciculus gracilis. Experimental Brain Research, 22, 471–493.
Apkarian, A. V., Bushnell, M. C., Treede, R. D., et al. (2005). Human brain mechanisms of pain perception and regulation in health and disease. European Journal of Pain, 9(4), 463–484.PubMed
Apkarian, A. V., & Hodge, C. J. J. (1989a). The primate spinothalamic pathways: I. A quantitative study of the cells of origin of the spinothalamic pathway. The Journal of Comparative Neurology, 288, 447–473.PubMed
Apkarian, A. V., & Hodge, C. J. J. (1989b). The primate spinothalamic pathways: II. The cells of origin of the dorsolateral and ventral spinothalamic pathways. The Journal of Comparative Neurology, 288, 474–492.PubMed
Apkarian, A. V., & Hodge, C. J. J. (1989c). Primate spinothalamic pathways: III. Thalamic terminations of the dorsolateral and ventral spinothalamic pathways. The Journal of Comparative Neurology, 288, 493–511.PubMed
Apkarian, A. V., & Shi, T. (1994). Squirrel monkey lateral thalamus. I. Somatic nociresponsive neurons and their relation to spinothalamic terminals. The Journal of Neuroscience, 14, 6779–6795.PubMed
Applebaum, A. E., Beall, J. E., Foreman, R. D., & Willis, W. D. (1975). Organization and receptive fields of primate spinothalamic tract neurons. Journal of Neurophysiology, 38, 572–586.PubMed
Armour, D. (1927). On the surgery of the spinal cord and its membranes. The Lancet, 2, 691–697.
Aziz, Q., Andersson, J. L., Valind, S., Sundin, A., Hamdy, S., Jones, A. K., et al. (1997). Identification of human brain loci processing esophageal sensation using positron emission tomography. Gastroenterology, 113, 50–59.PubMed
Baliki, M. N., Chialvo, D. R., Geha, P. Y., Levy, R. M., Harden, R. M., Parrish, T. B., & Apkarian, A. V. (2006). Chronic pain and the emotional brain: Specific brain activity associated with spontaneous fluctuations of intensity of chronic back pain. The Journal of Neuroscience, 26(47), 12165–12173.PubMed
Bennett, G. J., Nishikawa, N., Lu, G. W., Hoffert, M. J., & Dubner, R. (1984). The morphology of dorsal column postsynaptic (DCPS) spino-medullary neurons in the cat. The Journal of Comparative Neurology, 224, 568–578.PubMed
Bennett, G. J., Seltzer, Z., Lu, G. W., Nishikawa, N., & Dubner, R. (1983). The cells of origin of the dorsal column postsynaptic projection in the lumbosacral enlargements of cats and monkeys. Somatosensory Research, 1, 131–149.PubMed
Berkley, K. J. (1980). Spatial relationships between the terminations of somatic sensory and motor pathways in the rostral brainstem of cats and monkeys. I. Ascending somatic sensory inputs to lateral diencephalon. The Journal of Comparative Neurology, 193, 283–317.PubMed
Berkley, K. J., Guilbaud, G., Benoist, J., & Gautron, M. (1993). Responses of neurons in and near the thalamic ventrobasal complex of the rat to stimulation of uterus, cervix, vagina, colon, and skin. Journal of Neurophysiology, 69, 557–568.PubMed
Berkley, K. J., & Hubscher, C. H. (1995a). Are there separate central nervous system pathways for touch and pain? Nature Medicine, 1(8), 766–773.PubMed
Berkley, K. J., & Hubscher, C. H. (1995b). Visceral and somatic sensory tracks through the neuraxis and their relation to pain: Lessons from the rat female reproductive system. In G. F. Gebhart (Ed.), Visceral pain. Seattle: IASP Press.
Besson, J. M., & Chaouch, A. (1987). Peripheral and spinal mechanisms of nociception. Physiological Reviews, 67, 67–186.PubMed
Bielefeldt, K., Lamb, K., & Gebhart, G. F. (2006). Convergence of sensory pathways in the development of somatic and visceral hypersensitivity. American Journal of Physiology. Gastrointestinal and Liver Physiology, 291(4), G658–G665.PubMed
Bingham, B., Ajit, S. K., Blake, D. R., & Samad, T. A. (2009). The molecular basis of pain and its clinical implications in rheumatology. Nature Clinical Practice Rheumatology, 5(1), 28–37.PubMed
Birrell, G. J., McQueen, D. S., Iggo, A., & Grubb, B. D. (1993). Prostanoid-induced potentiation of the excitatory and sensitizing effects of bradykinin on articular mechanonociceptors in the rat ankle joint. Neurosciences, 54, 537–544.
Blair, R. W., Ammons, W. S., & Foreman, R. D. (1984). Responses of thoracic spinothalamic and spinoreticular cells to coronary artery occlusion. Journal of Neurophysiology, 51, 636–648.PubMed
Blair, R. W., Wenster, R. N., & Foreman, R. D. (1982). Responses of thoracic spinothalamic neurons to intracardiac injection of bradykinin in the monkey. Circulation Research, 51, 83–94.PubMed
Boivie, J. (1979). An anatomical reinvestigation of the termination of the spinothalamic tract in the monkey. The Journal of Comparative Neurology, 186, 343–370.PubMed
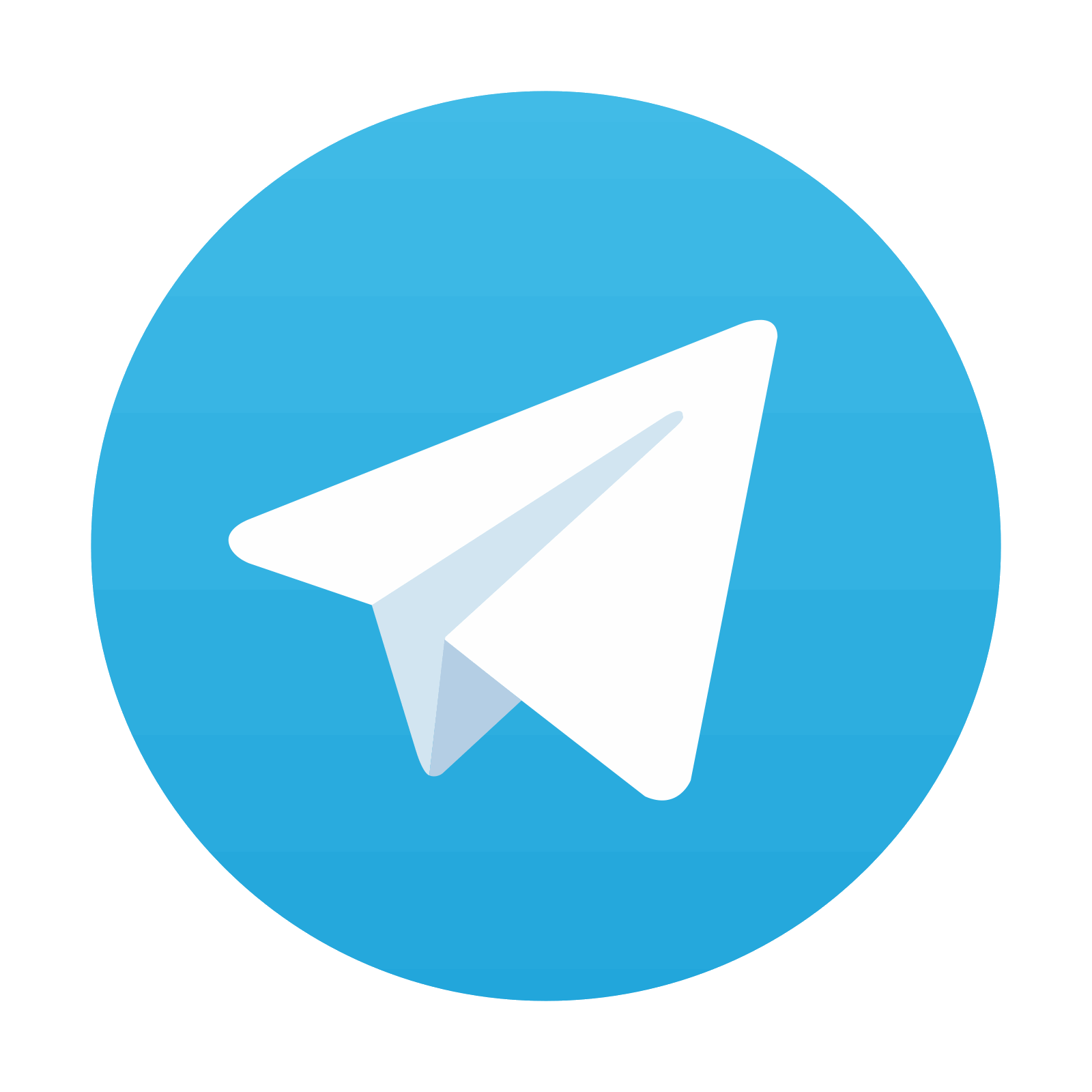
Stay updated, free articles. Join our Telegram channel
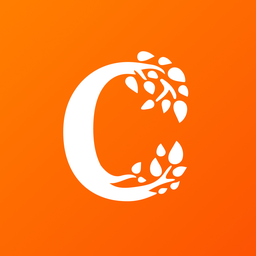
Full access? Get Clinical Tree
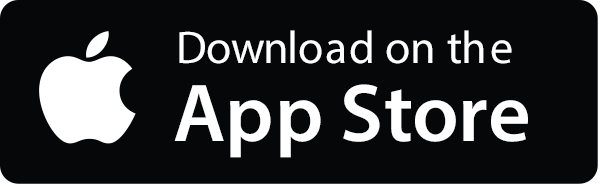
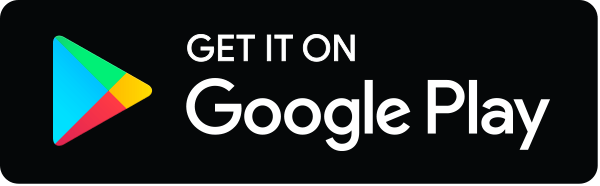