CHAPTER 17 Neonatology for Anesthesiologists
Advances in obstetrics and neonatology have greatly improved neonatal survival, especially for preterm neonates (Fig. 17-1). Because the survival rate of premature infants is on the rise, so is the need for anesthesia and surgery for these patients. To safely care for newborns in the operating room, anesthesiologists must appreciate and understand the enormous physiologic variability that exists among infants born between 23 and 44 weeks of gestation, so they can better understand how immature organs respond to surgery and anesthetic agents. Fetal development is also relevant, because fetal surgery is a reality in many medical centers, and providing anesthesia for midgestation fetuses (20 to 23 weeks’ gestation) via the mother poses a completely new set of challenges (Hirose et al., 2004). The purpose of this chapter is to present background information that will allow anesthesiologists to better understand the problems of neonates and to provide safe care for these patients based on an understanding of neonatal physiology. The preoperative evaluation of the neonate is arguably the most important part of anesthetic care.
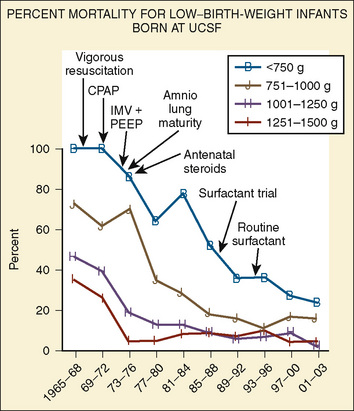
FIGURE 17-1 Percent mortality for low–birth-weight infants born at University of California, San Francisco.
Although some congenital anomalies are obvious at birth, others, such as gastrointestinal dysfunction, various central nervous system (CNS) malformations, or metabolic disorders, may not develop until later in life. In general, if one congenital anomaly is present, the anesthesiologist must be suspicious that there are others. Box 17-1 provides a list of disease entities and coexisting anomalies, and this list may be useful as part of a preoperative assessment (Jones and Pelton, 1976).
Box 17-1 Commonly Encountered Lesions in the Neonate
Development Of the Fetus and the Intrauterine Environment
The intrauterine environment has dramatic effects on growth and on the ability of neonates to adapt to extrauterine life. Although the impact of intrauterine events is most obvious during the first few hours and days of life, the effects of these events can last much longer. For example, phenytoin, thalidomide, alcohol, and maternal drug use has caused well-described syndromes, alterations in somatic growth, or drug-dependence and withdrawal (e.g., opioids or amphetamines). Exposure to drugs during pregnancy has been associated with anomalies of the heart, pulmonary circulation, brain, and other organs (e.g., cleft lip and palate) (Puhó et al., 2007).
However, the fetus is at a great disadvantage—it has no store of oxygen beyond that in its blood, because the lungs are filled with fluid and not gas (Dudenhausen et al., 1997). After only a minute of umbilical cord occlusion, the fetus’ Pao2 is less than 5 mm Hg. At the same time, the Paco2 steadily rises and the pH rapidly declines. The effects of intrauterine asphyxia can be sudden and profound, and they are often associated with severe hypoxia, hypotension, and acidosis (Fig. 17-2).
Labor and Delivery and Perinatal Events
The neonate’s respiratory rate increases briefly with the onset of asphyxia (see Fig. 17-2). Primary apnea quickly follows and lasts a variable amount of time. This is followed by slow gasping respirations and then by terminal apnea and death unless resuscitation is begun (Dawes, 1968). The heart rate and arterial blood pressure increase moderately, and bradycardia and hypotension follow. Because Cardiac Output (CO) is mostly dependent on heart rate in neonates, the severe, deep reduction in heart rate associated with asphyxia significantly reduces CO (Klopfenstein and Rudolph, 1978). To compensate for this reduction, blood flow is centralized to maintain blood flow and oxygen delivery to the brain, heart, and adrenal glands. The arterial blood pressure increases during gasping respirations and decreases markedly during terminal apnea. Heart rate, CO, and arterial blood pressure quickly improve with resuscitation. However, the metabolic component of acidosis may worsen as the pHa is corrected, because fixed acids are washed out of the periphery by the improved peripheral perfusion. If the pH does not improve with ventilation alone, an infusion of tromethamine (Tham) or sodium bicarbonate may be necessary. The lungs should be ventilated if sodium bicarbonate is administered, because each milliequivalent of sodium bicarbonate (when fully reacted with hydrogen ion) produces 25 cc of CO2 that must be removed via the lungs.
Labor and Delivery
Labor, fetal movements, and uterine contractions initiate removal of the 30 mL/kg of fluid that are normally present in the fetal lungs (Harding and Hooper, 1996). During labor, compression of the fetal chest by the uterus and vagina removes some of the lung fluid (Harding et al., 1990). After birth, breathing increases clearance of the lung fluid via the pulmonary capillaries and the lymphatics (see Pulmonary System, p. 518). The specific mechanism that initiates breathing at birth remains unclear, but several factors are known to play critical roles. First, placental factors that inhibit breathing in utero are removed by clamping the umbilical cord (Alvaro et al., 2004, 1997). Second, at birth the sudden release of the circumferential pressure around the chest allows the neonatal chest to recoil outward and draw gas into the lungs (Karlberg et al., 1962). Third, tactile stimulation and environmental thermal changes at birth stimulate breathing (Sinha and Donn, 2006). Lastly, the effects of hypoxia and hypercarbia on the chemosensors stimulate breathing (Taeusch et al., 2005).
Neonatal Asphyxia
Postnatal asphyxia is often the result of a continuum of intrauterine events, but it may also be caused by events that occur during labor and delivery. Immature respiratory control mechanisms can predispose neonates, especially premature neonates, to life-threatening responses to asphyxia. For example, the response to hypoxia during the first 3 to 4 weeks of life can be paradoxical, in that hypoxia produces a brief period of hyperpnea that is followed by bradypnea (Cross and Oppe, 1952; Brady and Ceruti, 1966). Hypothermia and hypercapnea blunt the initial hyperpnea (Ceruti, 1966; Rigatto et al., 1975). The ventilatory response to carbon dioxide increases with both postnatal and gestational age (see Chapter 3, Respiratory Physiology in Infants and Children) (Rigatto et al., 1975).
Although hypoxia may inflict long-term consequences on the fetus and newborn, hyperoxia can also cause significant morbidity, especially for preterm infants. For example, hyperoxia exposes preterm infants, especially those born before 32 weeks’ gestation, to a significant risk for retinopathy of prematurity (ROP; see below) and, in some cases, blindness (see Chapter 27, Anesthesia for Ophthalmic Surgery) (Sylvester, 2008). The normal Pao2 of a fetus is 20 to 30 mm Hg. After birth, a Pao2 of 60 mm Hg is probably hyperoxic for infants born at 24 to 36 weeks’ gestation. To avoid the effects of oxidative stress in the newborn, the oxygen saturation for premature infants is usually maintained between 88% and 93% (Pao2 of 45 to 60 mm Hg) in the NICU, and similar Sao2 levels are appropriate in the operating room. Continuous measurement of Sao2 makes it easier to maintain the desired oxygen saturation. Of note is one preterm infant, who never had an elevated Pao2 except in the operating room, but who developed ROP after surgery (Betts et al., 1977).
Metabolic Status: Early Postnatal Period
Thermal Environment
In a neutral thermal environment, neonates expend the least amount of energy to maintain their body temperature. The oxygen consumption (o2) of normal neonates is 6 cc/kg per minute, twice that of resting adults. During the first week of life,
o2 increases to about 10 cc/kg per minute and may be twice that during heat-related stress. Because of their high oxygen consumption, greater CO, and smaller reserve of oxygen (i.e., smaller functional residual capacity [FRC]), neonates, especially preterm neonates, develop hypoxia more quickly than adults. In fact, hypoxia may develop in as few as 10 seconds in apneic neonates, whereas it takes several minutes for this to occur in adults (Peabody et al., 1978a, 1978b).
Sodium, Calcium, and Glucose
Box 17-2 contains common fluid and electrolyte requirements for neonatal infants. Because premature, large-for-gestational age (LGA), small-for-gestational-age (SGA), and asphyxiated neonates are often hyper- or hypoglycemic, intravenous glucose may be required to maintain normoglycemia (serum glucose concentration of 40 to 90 mg/dL; see Glucose Metabolism, p. 537) (Jain et al., 2008). Similarly, calcium homeostasis is often difficult to achieve in sick infants (Bass and Chan, 2006). Initial calcium administration is usually based on normal neonatal requirements and on need, as determined by serial ionized calcium measurements. Calcium should be infused into a well-functioning intravenous catheter to avoid extravasation of calcium into the tissues and severe tissue necrosis. During surgery the calcium infusion site is usually under the surgical drapes and hard to see. Therefore, calcium should be infused into a reliable intravenous catheter, preferably a central venous catheter.
Box 17-2 Common Intravenous Fluid and Electrolyte Requirements in the Newborn
Sodium
Potassium
In full-term and near-term infants, sodium is seldom added to intravenous fluids during the first 24 hours of life. Urine output is low, despite the fact that 30 mL/kg of fluid must be removed from the lungs during and after birth (Herin and Zetterström, 1994). On the second day of life, maintenance sodium (2 to 4 mEq/kg per day) is added to the intravenous fluids to replace renal and gastrointestinal tract sodium losses, to compensate for abnormal fluid metabolism, or to counter the effect of diuretics (e.g., furosemide). In the extremely low-birth weight (ELBW) infant, fluid that contains sodium is often administered to maintain adequate intravascular volume, especially when transcutaneous fluid losses are excessive. After the first few days of life, an adequate sodium intake is essential for infants of all gestational ages to permit normal growth.
Gestational Age
The SGA Infant
In part, perinatal problems are related to gestational age and birth weight (Box 17-3). Infants who weigh less than the 10th percentile of weight for gestational age at birth are usually considered to be small for their gestational age. SGA infants have different problems from those of preterm infants (fewer than 37 weeks’ gestation) of the same weight (Lubchenco et al., 1963). Consequently, it is necessary to relate birth weight to gestational age. Although primary fetal (e.g., chromosomal disorders) and maternal (e.g., smoking or chronic disease) factors have been linked to intrauterine growth retardation, SGA is often the result of placental insufficiency. SGA newborns are often hypoglycemic because they have abnormally low glycogen stores. They commonly respond to placental insufficiency by increasing their red-blood-cell mass. Although an increase in red-blood-cell mass (polycythemia) increases the oxygen-carrying capacity of blood, polycythemia also has been associated with hyperviscosity, as well as renal failure, NEC, and CNS injury, especially if the hematocrit value exceeds 65% (Pallotto and Kilbride, 2006; Rosenberg, 2008). If the hematocrit value is higher than 65% in well-hydrated neonates, an exchange transfusion should be considered to reduce the hematocrit level to 55% or lower before surgery, although there is no evidence that doing so for patients who are not having surgery improves outcome (Dempsey and Barrington, 2006). However, the combination of polycythemia, hypotension, and dehydration that are common in sick SGA infants during anesthesia and surgery may increase their risk for hyperviscosity-related problems. Arterial blood pressure should be kept normal or slightly elevated throughout surgery. SGA infants may be relatively dehydrated at birth and usually do not lose the normal 200 to 300 g of weight that appropriate-for-gestational-age (AGA) neonates lose over the first 2 to 3 days of life. SGA neonates usually gain weight from birth. In spite of their low birth weights, the heart rates and blood pressures of SGA infants are similar to those of AGA infants of similar gestational ages. SGA neonates have similar incidences of hyaline membrane disease (HMD) to AGA babies of the same gestational age.
The LGA Infant
LGA infants are prone to birth injuries (e.g., fractures or intracranial bleeds) (Lawrence, 2007). Those born to diabetic mothers (a common reason for LGA) may have difficulty maintaining normal blood glucose concentrations and glucagon may be required to do so (Van Howe and Storms, 2008). On occasion, tracheal intubation may be difficult in LGA babies because of fat deposits in their mouth, tongue, and neck. Finding adequate insertion sites for intravenous fluid may be difficult because the veins are buried in tissue. It may be necessary to insert a central venous catheter or an external jugular vein catheter to ensure adequate intravenous access.
The Premature Infant
Prematurity is defined as birth before 37 weeks of gestation. Premature infants account for 12.8% of all births in the United States (646,047 of 4,265,996), and the incidence has increased 21% since 1990 (Martin et al., 2008). The increase has been most dramatic between the gestational ages of 34 to 36 weeks (Davidoff et al., 2006). While the rates of VLBW (fewer than 1500 g) and ELBW (fewer than 1000 g) deliveries remained relatively unchanged from 2005 to 2006, the percentage of moderately low birth weight (1500 to 2499 g) deliveries increased slightly. In 2005, short gestation and low birth weight was the second leading cause of infant death (16.5%), ranked only below congenital malformations (19.5%) (Martin et al., 2008). However, in non-Hispanic black, and Puerto Rican infants, disorders related to short gestation and low birth weight were the leading causes of death. Today, the Centers for Disease Control (CDC) recognize prematurity, not birth defects, as the leading cause of infant mortality in the United States (Andrews et al., 2008).
Late Preterm (35 to 37 Weeks’ Gestation)
In the absence of congenital anomalies or perinatal asphyxia, infants born between 35 and 37 weeks’ gestation usually do well and have few of the common medical or surgical problems found in more premature infants. However, late preterm birth is associated with increased mortality (threefold higher than term infants), respiratory distress or apnea requiring support, and rehospitalization (Escobar et al., 2006; Tomashek et al., 2007). In addition, sepsis and intrapartum complications, centered on the placenta and cord, have contributed to increased mortality in late preterm infants (Tomashek et al., 2007). In addition, late-preterm infants may have some difficulty attaining full feeds, and the incidence of hyperbilirubinemia (not associated with Coombs’ positivity or ABO/Rh incompatibility) occurs more commonly in late preterm infants than in term infants. Late-preterm infants born to diabetic mothers may have pulmonary immaturity and respiratory distress syndrome (RDS) (Deorari et al., 1991). Late-preterm infants born to nondiabetic mothers usually have similar lung function to that of term infants. Most surgical intervention in this group of patients is for treatment of congenital anomalies.
The rate of late preterm birth has increased 25% since 1990, and attention has been drawn to the increased mortality associated with late-preterm compared with term gestation (Escobar et al., 2006; Shapiro-Mendoza et al., 2006; Engle et al., 2007; Tomashek et al., 2007; Martin et al., 2008; McIntire and Leveno, 2008). The morbidity and mortality rates of late-preterm infants are particularly noticeable because of the large number of births at this developmental stage; in 2006, late-preterm births accounted for more than 71% of all preterm births (9.14%) (Martin et al., 2008). However, the long-term outcome of this group has not been well documented, in part related to the longstanding view of this group as “near term” rather than late preterm (Jain, 2007).
30 to 34 Weeks’ Gestation
Before the introduction of exogenous pulmonary surfactant into clinical practice, this group of infants often had RDS, also known as HMD (see Respiratory Distress Syndrome, p. 520). Complications of their respiratory care, including pneumothorax, pulmonary interstitial emphysema, chronic lung disease (CLD), and blood loss from repeated blood sampling, were common. Treatment of RDS with surfactant has dramatically reduced the incidence and severity of this disease and it complications. The sequelae of pulmonary immaturity and of the supportive care used to treat lung immaturity are fewer and less severe since the introduction of exogenous surface-active material (SAM). However, a new form of bronchopulmonary dysplasia (BPD), called new BPD, occurs in small preterm infants (Bancalari, 2001; Bancalari and del Moral, 2001). Approximately 20% to 25% of these infants have some form of CLD.
Although uncommon in infants after 34 weeks’ gestation, newborns with a gestational age of 30 to 32 weeks often demonstrate temperature instability, especially if they are septic or asphyxiated. Feeding intolerance is also common, and it may take weeks before effective oral feeding and consistent weight gain occur. Before adequate enteral feeds are established, infants born at 30 to 34 weeks’ gestation are often hypocalcemic or hypoglycemic. They are also prone to NEC, especially if they have a large patent ductus arteriosus (PDA) with significant left-to-right shunting of blood (Kitterman, 1980). Because enteral feeds must be advanced slowly, peripheral or central intravenous alimentation may be required for some time. Parenteral nutrition, for even 1 to 2 weeks, can cause hepatic and renal toxicity (Moreno Villares, 2008).
The incidence of a PDA is high (20% to 30%) in infants of this gestational age, but the hemodynamic consequences are often mild. Their PDA commonly closes spontaneously. If it does not close, chemical (with indomethacin) or surgical closure of the PDA may be necessary. The incidence of intracerebral hemorrhage (ICH) is higher in this age group and increases with decreasing gestational age, sepsis, asphyxia, or precipitous birth. Apnea is also more common at this developmental stage, especially in those infants who have sepsis, temperature instability, metabolic abnormalities (e.g., hypoglycemia or hypocalcemia), or anemia (Theobald et al., 2000).
Fewer than 26 Weeks’ Gestation
In this group of patients, the CNS is fragile, and ICH is common (Kadri et al., 2006). The severity of ICH is a common determinant of an infant’s prognosis and long-term outcome, and it correlates with later neurologic injury (see The Central Nervous System, p. 528). Apnea and perioperative pulmonary insufficiency occur often and may persist for months. From the first few weeks to the first months of life, most infants of this developmental stage require mechanical ventilation, continuous positive airway pressure (CPAP), or an increased inspired oxygen concentration after surgery. Ventilatory support is often required, even if these interventions were not necessary preoperatively. Prolonged tracheal intubation is occasionally required and may lead to subglottic stenosis and lower airway obstruction.
Review of Systems and Developmental Physiology
Head and Neck
Anatomic differences of the head and neck between neonates and older patients make the management of the neonate’s airway challenging (Dickison, 1987). The cricoid ring is the narrowest portion of the neonate’s upper airway because the larynx has been thought to be cone shaped, rather than cylindrical as it is after 5 years of age (Coté et al., 1993). However, this concept has been challenged (Litman et al., 2003; Dalal et al., 2009; Motoyama, 2009). These newer studies indicate that the shape of the larynx is cylindrical, as it is in adults, but the cricoid ring remains the fixed and narrowest point of the upper airway system.
The narrowest portion of the cone is the cricoid ring. This is, in part, because the neonate’s posterior larynx is more cephalad than the anterior larynx. When looking from above, the cricoid ring is really an ellipse and not a circle. By 5 years of age, the posterior larynx descends, the cricoid ring is circular, and the vocal cords are the narrowest portion of the airway (as they are in adults). These anatomic differences place the neonatal larynx in a more cephalad position than it is later in life. The epiglottis is relatively large, which makes it more difficult to lift with a laryngoscope blade. In fact it is easier to use a straight laryngoscope blade like a curved blade, place the tip in the vellecula, and pull up and out at a 45-degree angle to bring the glottic opening into view. The cephalad larynx and relatively large tongue also make it more difficult to maintain an airway or to intubate the trachea. However, the neonate’s large occiput naturally places the head (when looking straight forward) in the “sniffing” position (i.e., an optimal position to ventilate the lungs and intubate the trachea). Extension or flexion of the head usually makes it more difficult to bag-and-mask ventilate the lungs and to intubate the trachea. A small jaw (micrognathia) or receding jaw (e.g., Pierre-Robin or Treacher-Collins syndromes) may make it difficult or impossible to directly visualize the vocal cords. A large tongue (as with hypothyroidism, Down syndrome, or Beckwith-Wiedemann syndrome) can also make tracheal intubation difficult or impossible. Techniques other than direct laryngoscopy may be needed to intubate the trachea of these infants (see Chapter 12, Airway Management).
Cleft lip, with and without a cleft palate, occurs in 1:600 to 1000 live births and is associated with other congenital defects in 13% to 50% of patients (see Chapter 25, Anesthesia for Plastic Surgery) (Arosarena, 2007). Tracheal intubation is occasionally more difficult in patients with a cleft palate because it may be difficult to fix the patient’s tongue against the palate with the laryngoscope blade. If the tongue cannot be fixed against the palate, it may flop over the laryngoscope blade and block the view of the glottis. Choanal atresia occurs in 1:7000 to 1:8000 live births and is often diagnosed in the delivery room when a catheter cannot be passed through a nostril into the pharynx or when the neonate cannot breath and becomes cyanotic and bradycardic when the mouth is closed and the airway is totally obstructed (see Chapter 12, Airway Management) (Samadi et al., 2003). Thus, when using bag-and-mask ventilation on patients who have choanal atresia, the mouth must be kept open or an oral airway must be inserted. Some patients who have choanal atresia have the CHARGE association, which is defined through the anacronym as follows: C, Colobomatous malformation; H, Heart defect; A, Atresia choane; R, Retardation; G, Growth deficiency/Genital hypoplasia; and E, Ear anomalies (Sanlaville and Verloes, 2007).
The Pulmonary System
Development
Lung development is divided into the following five stages:
The latter stage begins at about 36 weeks’ gestation and continues until at least 18 months of age (Langston et al., 1984; Kotecha, 2000a, 2000b; Joshi and Kotecha, 2007). Knowledge of these phases of lung development allows estimation of when the various lung malformations occur in utero. For example, malformations of the conducting airways (e.g., cystic adenomatoid malformation) occur before 16 weeks’ gestation. Upper airway abnormalities occur by 6 weeks’ gestation, bronchial malformations between 6 and 16 weeks of gestation, and lung hypoplasia after 16 weeks’ gestation. In general, extrauterine viability is increased after 26 weeks’ gestation, because the respiratory saccules have developed and the capillaries are in close approximation to the developing distal airways. Before this time, both the vascular network and surface area in the lungs may be inadequate for gas exchange. However, in the past decade many babies have survived after 24 to 25 weeks’ gestation, usually with enormous effort and cost; these extremely premature infants often survive with severe CLD (Langston et al., 1984).
Most alveoli develop after birth, increasing from 20 million terminal air sacs at term to about 300 to 400 million alveoli at 18 months of age (Thurlbeck, 1982; Kotecha, 2000a, 2000b). Specific cell types are not recognizable in the lung until the canalicular stage of development (17 to 28 weeks’ gestation). During the last 10% to 20% of gestation, type II pneumocytes are more commonly identified. Although present in the human fetus by 22 weeks’ gestation, type II pneumocytes are more prominent after 34 to 36 weeks of gestation. The major distinguishing feature of type II cells is the presence of osmophilic lamellar bodies, which correlate closely with the presence of SAM in the lungs (Kotecha, 2000a, 2000b). The alveolar-capillary barrier is formed by type I pneumocytes (see Chapter 3, Respiratory Physiology in Infants and Children).
By 16 weeks’ gestation, all subdivisions of the conducting airways have formed, including the main-stem bronchi and the conducting and terminal bronchioles. Preterm infants often require mechanical ventilation or CPAP and supplemental oxygen, which interfere with the normal, complex process of lung and airway development. The barotrauma, oxidative stress, and inflammatory injury associated with these life-saving interventions and the effects of superimposed infections predispose preterm infants to airway and lung injury and to abnormal airway resistance and reactivity (Van Marter, 2005).
The diaphragm arises in part from the third through fifth cervical somites, and myoblasts from these somites migrate into the septum transversum to form diaphragmatic muscle (Mitchell and Sharma, 2009). The central tendon of the diaphragm arises from the mesoderm of the septum transversum. Somites of the lateral chest wall form the lateral portions of the diaphragm. The nerve supply of the diaphragm is from the third through fifth cervical somites. Bilateral pericardioperitoneal canals, located lateral to the developing diaphragm, cause a Bochdalek hernia if the canals fail to close. Complete closure of the diaphragm normally occurs at 10 to 12 weeks’ gestation, when the bowel is returning to the abdominal cavity from the amnion, where it resides in early gestation. If the diaphragm is incompletely formed, the returning bowel follows the path of least resistance and enters the chest. When this occurs lung growth ceases on the ipsilateral side. The mediastinum is then pushed into the opposite chest by the bowel and abdominal organs and contralateral lung growth is also reduced. Consequently, neonates who have a left-sided diaphragmatic hernia must survive with approximately two thirds of one lung or less (de Lorimier et al., 1967). In some cases, the amount of lung present is insufficient for survival (see Chapter 18, Anesthesia for General Surgery in the Neonate).
Failure of the lung buds to separate from the foregut causes a tracheal esophageal fistula (see Chapter 18, Anesthesia for General Surgery in the Neonate). The connection usually is found between the distal esophagus and the trachea, just above the carina. Failure of the distal and proximal ends of the esophagus to connect with each other results in esophageal atresia. Patients with esophageal atresia usually have copious secretions in the proximal esophageal pouch, and placing the patient flat without removing the secretions may cause aspiration of the secretions. Placing a suction catheter in the proximal esophageal pouch and connecting it to suction reduces this likelihood.
Early Postnatal Period: Changes in Oxygenation and Pulmonary Vascular Resistance
Although removal of fluid from the fetal lung begins during labor and delivery, the major transition to an air-filled lung occurs immediately after birth. The first active inspiration after birth produces a negative intrapleural pressure of 80 to 100 cm H2O, which is required to overcome the high surface tension, low compliance, and high resistance of the liquid-filled lungs (Scarpelli and Mautone, 1984; Vyas et al., 2005). These pressures are not necessary when artificially ventilating neonatal lungs, however. In fact, delivering large tidal volumes and high airway pressures injure neonatal lungs (Bjorklund et al., 1997). In full-term neonates, the initial few spontaneous breaths are of 20 and 80 cc O2 each. A small fraction of this volume is expired. The gas remaining in the lung forms the residual volume (RV) and the FRC, which are required for adequate gas exchange (Avery, 1974). If removal of lung fluid is delayed (e.g., with cesarean section) transient tachypnea of the newborn (TTN) develops (Guglani et al., 2008). Neonates with TTN breathe 60 to 150 times a minute and are often hypoxic and hypercarbic. Many of them require supplemental oxygen, and some require mechanical ventilation. Diuretics fail to induce a diuresis and improve lung function until a spontaneous diuresis occurs several days after birth (Lewis and Whitelaw, 2002).
Labor reduces pulmonary vascular resistance (PVR) by approximately 10%, but the most significant decrease occurs with the onset of breathing (Bland, 1983). Lung expansion increases alveolar and arterial Po2, which dilates the pulmonary arterioles, decreases PVR and increases pulmonary blood flow (Dawes, 1974). These changes in PVR and blood flow are critical for conversion of the fetal circulation to the adult form of circulation (see Chapters 3, Respiratory Physiology, and 4, Cardiovascular Physiology). That is, transition to the gas-filled lung at birth, increased Fio2 (air), and increased pH (decreased Paco2) all interact to reduce PVR and increase pulmonary blood flow. Over the next 1 to 2 months, PVR decreases to adult levels. However, infants with cyanotic congenital heart disease (CHD) or large left-to-right shunts may have elevated PVR for months or longer (Rudolph, 2007).
The dramatic rise in pulmonary blood flow at birth increases the left atrial pressure and functionally closes the foramen ovale, preventing right-to-left shunting of deoxygenated blood through this structure. An increase in systemic vascular resistance that exceeds PVR dramatically reduces right-to-left blood flow through the ductus arteriosus. The rise in arterial oxygen tension at birth constricts and physiologically closes the ductus arteriosus in full-term but not in preterm neonates (Clyman et al., 1978). Nitric oxide (NO) and prostaglandins are important for anatomic closure of the ductus arteriosus, which usually occurs by 2 to 3 weeks of age (Born et al., 1956; Seidner et al., 2001; Clyman, 2006). If during this time hypoxia, acidosis, cold, or excessive airway pressures are present, the right atrial pressure increases and right-to-left shunting of blood can occur at both the atrial and the DA levels. When right-to-left shunting of blood occurs, hypoxemia ensues. The resulting pulmonary blood flow pattern mimics the pattern present in utero and is often referred to as persistent fetal circulation (PFC).
Establishing normal pulmonary blood flow and ventilation increases both arterial and mixed venous oxygen tensions. Although the transition to air breathing increases the oxygen content of blood, the Pao2 of the neonate is usually 55 to 85 mm Hg for the first month of life, rather than the 95 to 100 mm Hg present after that. The lower Pao2 is the result of a very compliant, cartilaginous neonatal chest wall that does not recoil outward at end-expiration, as it does in older children and adults (Koch and Wendel, 1968; Davis and Bureau, 1987). Failure to recoil outward produces a transpulmonary (intrapleural) pressure of 0 cm H2O—not −5 cm H2O pressure, as occurs in older patients. Because negative intrapleural pressures are important for maintenance of a normal FRC, neonates are predisposed to atelectasis and a lower Pao2. In fact, application of sufficient negative pressure around the neonate’s chest to produce a pleural pressure of −5 cm H2O, increases the Pao2 from about 65 mm Hg to nearly 100 mm Hg, indicating that the negative intrapleural pressure expands the lungs, increases FRC, reduces atelectasis, and improves the match of ventilation to perfusion (Thibeault et al., 1968). Application of negative intrapleural pressure, positive end expiratory pressure (PEEP), or CPAP also reduces the amount of atelectasis and improves oxygenation (Gregory et al., 1975).
In addition to its effects on transpulmonary pressure, the newborn’s compliant rib cage has another unwanted effect on ventilation. That is, the cartilaginous, compliant chest wall tends to collapse inward (retract) during spontaneous inspiration, causing paradoxic chest-wall motion and limiting airflow (Knill et al., 1976). The circular configuration of the rib cage (rather than the ellipsoid rib cage of adults) and the horizontal angle of insertion of the diaphragm (rather than the oblique angle in adults) also distort the rib cage and make the diaphragm less efficient (Muller and Bryan, 1979).
Other factors also affect the infant’s work and efficiency of breathing. For instance, the full-term infant’s diaphragm is comprised of only 25% fatigue-resistant, slow-twitch, highly oxidative type I fibers, and the preterm infant has only 10% (the adult diaphragm has 55%). The lower percentage of type I fibers predisposes the diaphragm to fatigue (Keens et al., 1978). The intercostal muscles show a similar developmental pattern. Expression of various isoforms of the myosin heavy chains in muscle fibers of the diaphragm and the intercostal muscles of newborn and young infants may contribute to easier fatigability (Watchko and Sieck, 1993; Zhan et al., 1998). Less effective force-frequency, length-tension, and force-velocity relationships and a mismatch of energy supply and demand characterize diaphragmatic muscle and predisposes these newborns to fatigue, especially when there is widespread atelectasis (e.g., RDS) or poor chest wall compliance (e.g., anasarca) (Watchko et al., 1986; Watchko and Sieck, 1993). Methylxanthines improve neonatal diaphragmatic function (Maycock et al., 1992).
Healthy neonates breathe 30 to 60 times per minute to maintain normal oxygenation and to remove the increased amount of CO2 produced by an oxygen consumption of 6 to 10 cc/kg per minute. This respiratory rate also helps maintain the FRC by reducing the amount of time available for expiration and by trapping gas within the lung. At normal respiratory rates, the time constant of the lungs (compliance of lungs × airway resistance [C• × Raw]) is, on average, 0.25 seconds. A slow respiratory rate, apnea, or bradypnea can reduce the FRC, especially in preterm neonates (Fig. 17-3). A low level of PEEP (5 to 7 cm H2O) should always be added to maintain the FRC of infants and young children, especially when slow ventilatory rates are used.
Naturally occurring SAM or pulmonary surfactant is by far the most important mechanism for keeping alveoli of different sizes or diameters open. Initially, SAM appears in fetal lungs by 20 weeks’ gestation, even though no functional alveoli exist (Clements, 1961). At about 35 to 36 weeks’ gestation, the amount of SAM increases and is secreted onto the alveolar surface. The pulmonary surfactant monolayer creates a gas-liquid interface on the alveolar surface and drastically decreases surface tension during exhalation as the surface area decreases. During inspiration the monolayer is thinned, and the surface tension increases. SAM keeps the surface tension relatively constant regardless of the changes in the surface area and the radius of the alveoli. In neonates with RDS, there is loss of or inactivation of surfactant, and surface tension increases. The variability of surface tension normally occurring with changes in surface area is lost; alveoli develop higher pressures, collapse during exhalation, and remain collapsed (see Chapter 3, Respiratory Physiology in Infants and Children).
An additional mechanism that helps keep the alveoli open is the interdependence among alveoli, small airways, and lung parenchyma. Because the alveoli are attached to each other by connective tissues, the tendency of one alveolus to collapse during expiration pulls its neighbor open (Takishima and Mead, 1972). Millions of alveoli exerting this “pulling” effect help maintain FRC. Furthermore, blood-filled capillaries act as an exoskeleton for alveoli. If the pulmonary blood volume is inadequate, atelectasis develops. Increasing the blood volume increases FRC (Table 17-1) (Gregory, personal observation).
TABLE 17-1 Changes in FRC Associated with Blood Transfusion in Five Term Infants*
Functional Residual Capacity (FRC) mL/kg | Blood Transfused mL/kg |
15 ± 5 | 0 |
22 ± 4 | 5 |
27 ± 6 | 15 |
Gregory GA: Unpublished data.
* FRC was measured by helium dilution.
Surface Active Material
The demonstration by Avery and Mead (1959) that surfactant deficiency is the primary cause of RDS eventually led Phibbs et al. (1991) and Fujiwara et al. (1990) to use commercially available surfactant prophylactically after birth or in the rescue mode after the symptoms of RDS were apparent. Intubated infants of fewer than 28 weeks’ gestation often receive 1 or 2 doses of surfactant 12 hours apart. During preoperative evaluation of a newborn with RDS, it should be determined when the last dose of SAM was given and if an additional dose is required before surgery because of progressive atelectasis or worsening blood gases and oxygen requirement.
Pulmonary gas exchange is only possible after a significant portion of the lung fluid present in utero is removed from the airways and alveoli. Increased pulmonary lymphatic flow and surface-active phospholipids and proteins facilitate this process (Humphreys et al., 1967; King, 1982). Surfactant reduces the surface tension of the gas-liquid interface of the alveoli, keeping the air spaces open at end expiration. This reduction of surface tension is critical for the maintenance of FRC and gas exchange.
Type I pneumocytes account for more than 90% of the alveolar surface cells; type II pneumocytes, the SAM-storing cells, populate most of the remaining surface. The total weight of SAM consists of about 10% protein, 90% lipids, and 0.1% carbohydrates. The lipids lower the surface tension, but the proteins allow absorption and dispersion of the lipids in the air-liquid interface. Proteins are also necessary for reuptake of SAM by type II cells. Congenital absence of these proteins leads to a chronic RDS-like state (Yurdakök, 2004). During inspiration SAM is spread over the alveolar surface. During expiration it is compacted to lower the alveolar surface tension. SAM also stabilizes small airways and is important for pulmonary defense mechanisms (Jarstarand, 1984).
Respiratory Distress Syndrome
Neonatal RDS occurs when the quantity of SAM is insufficient or when there is delayed synthesis or release of surfactant (e.g., in infants of diabetic mothers). Giving betamethasone to the fetus, by way of the mother, before 36 weeks’ gestation accelerates the production and release of SAM from the type II alveolar epithelial cells and reduces the incidence of RDS (Liggins and Howie, 1972). Typical clinical features of RDS include: early onset of tachypnea; intercostal, sternal, and suprasternal retractions; grunting respirations; oxygen desaturation; respiratory and metabolic acidosis; and death if not treated. A chest radiograph shows a diffuse reticulogranular pattern and air bronchograms (Fig. 17-4). Unless SAM is given, the natural course of RDS includes worsening symptoms for 2 to 3 days, resulting in either improvement or death. In some cases, respiratory function may deteriorate, rather than improve, because of a superinfection or complications of ventilatory support. RDS-associated death is often the result of severe lung injury induced by mechanical ventilation, sepsis, renal or hepatic failure, or CNS injury. This severe clinical pattern occurs less commonly when pulmonary surfactant is provided early. About 5% of all babies with RDS are born at term.
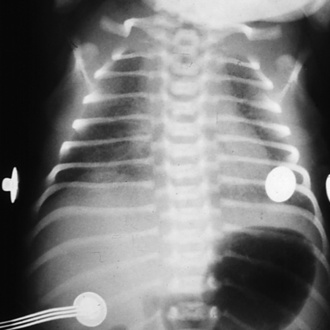
FIGURE 17-4 RDS. Note the ground-glass appearance and the presence of air bronchograms.
(From Brozanski BS, Bogen DL: Neonatology. In Zitelli BJ, Davis HW, editors: Atlas of pediatric physical diagnosis, ed 4, Philadelphia, 2002, Mosby.)
Before the development of commercially available surfactant, lung injury (e.g., pneumothorax, pulmonary interstitial emphysema, or BPD), blood loss from repeated blood sampling, and sepsis and its secondary effects (e.g., renal and hepatic dysfunction) were relatively common. Since 1990, exogenous SAM administration has significantly reduced the need for mechanical ventilation and oxygen therapy in preterm infants because of its effects on lung mechanics, including distensibility of the lung and end-expiratory volume stability (Morley et al., 2008). However, volutrauma (high tidal volume), and barotrauma (high airway pressure) persist in causing lung injury despite recent decreases in the incidence and severity of classic RDS and a reduction in the complications of pulmonary immaturity and supportive care. Despite all of these changes, the incidence of BPD has remained stable at about 20% (higher in ELBW infants) over the last 20 years (Bancalari and del Moral, 2001). But the BPD now seen in surfactant-treated lungs is different from classic BPD and is called new BPD. New BPD most commonly develops in ELBW infants, is characterized by a more uniform pattern of injury, and seems to represent an arrest of lung growth. Persistence of BPD, in spite of surfactant, suggests that other factors beside SAM deficiency contribute to BPD (Bancalari, 2001). Inflammatory and oxidative injury caused by infection, oxygen therapy, and “gentle” ventilation during periods of rapid lung growth and development, especially in the ELBW infants, seem to cause long-term pulmonary dysfunction (Kramer et al., 2009). The increased number of survivors has enlarged the number of patients (with and without BPD) requiring surgical treatment for coexisting long-term sequelae of prematurity.
Recent efforts to minimize lung injury caused by mechanical ventilation of premature infants have included introducing nasal CPAP (nCPAP) in the delivery room to prevent or treat RDS (Morley et al., 2008). This tactic avoids endotracheal intubation in patients and has markedly reduced the use of SAM. On the other hand, some infants with RDS (especially those weighing less than 1000 g) often fail a trial of nCPAP and require endotracheal intubation. They are usually treated with surfactant when their trachea are intubated.
Oxidative Injury in the Newborn
Although the role of oxygen in ROP is well established, oxygen toxicity of other organs, especially those of more mature newborns, is being increasingly recognized. Despite its widespread use for more than a century and the recognition that oxygen can be toxic for at least 50 years, the precise molecular mechanism of oxygen toxicity remains unknown (Tin, 2002). The severity of BPD has been correlated with oxygen exposure and with the oxygen saturation level used. In addition, greater oxidative injury to the heart, brain, and kidneys has been reported when neonatal resuscitation is accomplished with supplemental oxygen (Munkeby et al., 2004; Martín et al., 2007).
ROP is a multifactorial disease, but two main factors are associated with its development—prematurity and oxygen (see Chapter 27, Anesthesia for Ophthalmic Surgery). As stated, the neonate is exposed in utero to an oxygen tension of 20 to 30 mm Hg. Birth raises the Pao2 to 55 to 85 mm Hg that may represent hyperoxia, especially for preterm neonates. The retinal vessels grow radially outward from the center of the optic disc from about 16 to 40 weeks’ gestation. Neonates born before term have incompletely developed retinal vessels (Roth, 1977). Two phases of ROP have been described. The initial phase, which occurs between 30 and 32 weeks’ gestation, is associated with inhibition of growth and loss of retinal vessels. Growth of the retina under these circumstances leads to retinal hypoxia. The second phase of ROP is associated with effusive retinal neovascularization and occurs between 32 and 34 weeks’ gestation. New vessels form in the avascular regions and send up fibrous bands to the vitreous gel and lens. These strands are responsible for retinal detachment in some premature infants (Chen and Smith, 2007). Vascular endothelial cell growth factor (VEGF) is required for new vessel growth in utero and afterwards. Administering oxygen after birth decreases VEGF during the first phase of ROP, which decreases vessel growth. In the second phase of ROP, retinal hypoxia increases VEGF expression, which causes exuberant vessel overgrowth. Insulin-like growth factor-1 (IGF-1) is required for VEGF to have its maximal effect. During gestation, serum concentrations of IGF-1 increase, and the severity of ROP is directly correlated with the level of IGF-1. In utero, IGF-1 is supplied by both the placenta and the amniotic fluid. This is withdrawn at birth, decreasing the effectiveness of VEGF, and vessel growth decreases. Excessive amounts of oxygen turn off VEGF. Thus, both VEGF and IGF-1, under appropriate circumstances, inhibit vessel growth and cause vessel regression. Because of the severe retinal hypoxia in phase I of ROP, VEGF and IGF-1 concentrations increase. Retinal vessels’ growth causes the severe problems seen in the later stages of ROP. Avoidance of excessive oxygen concentrations in the operating room is therefore necessary (Betts et al., 1977).
Because there is evidence that supplemental oxygen causes a significant amount of injury, the use of supplemental oxygen should be limited to that required to maintain the oxygen saturation between 85% and 93%, both during resuscitation at birth and during the entire neonatal period (Rabi et al., 2007).
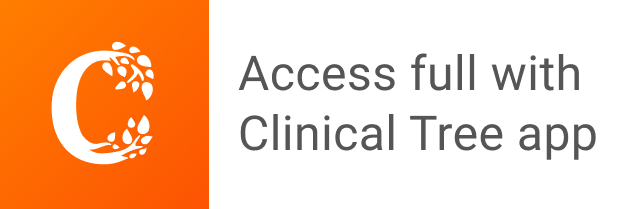