Muscle Function and Neuromuscular Blockade
At first, muscle relaxants were used only occasionally, in small doses, as an adjuvant to aid in the management of a difficult case; they were not used routinely. A tracheal tube was not always used, the lungs were not ventilated artificially and residual block was not routinely reversed; all of these caused significant morbidity and mortality, as demonstrated in the retrospective study by Beecher & Todd (1954). By 1946, however, it was appreciated that using drugs such as curare in larger doses allowed the depth of anaesthesia to be lightened, and it was suggested that incremental doses should also be used during prolonged surgery, rather than deepening anaesthesia – an entirely new concept at that time. The use of routine tracheal intubation and artificial ventilation then evolved.
PHYSIOLOGY OF NEUROMUSCULAR TRANSMISSION
Acetylcholine, the neurotransmitter at the neuromuscular junction, is released from presynaptic nerve endings on passage of a nerve impulse (an action potential) down the axon to the nerve terminal. The neurotransmitter is synthesized from choline and acetylcoenzyme A by the enzyme choline acetyltransferase and stored in vesicles in the nerve terminal. The action potential depolarizes the nerve terminal to release the neurotransmitter; entry of Ca2 + ions into the nerve terminal is a necessary part of this process, promoting further acetylcholine release. On the arrival of an action potential, the storage vesicles are transferred to the active zones on the edge of the axonal membrane, where they fuse with the terminal wall to release the acetylcholine (Fig. 6.1). Three proteins, synaptobrevin, syntaxin and synaptosome-associated protein SNAP-25, are involved in this process. These proteins along with vesicle membrane-associated synaptotagmins cause the docking, fusion and release (exocytosis) of acetylcholine from the vesicles. There are about 1000 active sites at each nerve ending and any one nerve action potential leads to the release of 200–300 vesicles. In addition, small quanta of acetylcholine, equivalent to the contents of one vesicle, are released at the neuromuscular junction spontaneously, causing miniature end-plate potentials (MEPPs) on the postsynaptic membrane, but these are insufficient to generate a muscle action potential.
FIGURE 6.1 The neuromuscular junction with an axon terminal, containing vesicles of acetylcholine. The neurotransmitter is released on arrival of an action potential and crosses the junctional cleft to stimulate the postjunctional receptors on the shoulders of the secondary clefts. (Reproduced with kind permission of Professor WC Bowman.)
The active sites of release are aligned directly opposite the acetylcholine receptors on the junctional folds of the postsynaptic membrane, lying on the muscle surface. The junctional cleft, the gap between the nerve terminal and the muscle membrane, has a width of only 60 nm. It contains the enzyme acetylcholinesterase, which is responsible for the ultimate breakdown of acetylcholine. This enzyme is also present, in higher concentrations, in the junctional folds in the postsynaptic membrane (Fig. 6.1). The choline produced by the breakdown of acetylcholine is taken up across the nerve membrane to be reused in the synthesis of the transmitter.
The nicotinic acetylcholine receptors on the postsynaptic membrane are organized in discrete clusters on the shoulders of the junctional folds (Fig. 6.1). Each cluster is about 0.1 μm in diameter and contains a few hundred receptors. Each receptor consists of five subunits, two of which, the alpha (α; MW = 40 000 Da), are identical. The other three, slightly larger subunits, are the beta (β), delta (δ) and epsilon (ε). In fetal muscle, the epsilon is replaced by a gamma (γ) subunit. Each subunit of the receptor is a glycosated protein – a chain of amino acids – coded by a different gene. The receptors are arranged as a cylinder which spans the membrane, with a central, normally closed, channel – the ionophore (Fig. 6.2). Each of the α subunits carries a single acetylcholine binding region on its extracellular surface. They also bind neuromuscular blocking drugs.
FIGURE 6.2 Two postjunctional receptors, embedded in the lipid layer of the postsynaptic muscle membrane. The α, β, ε and δ subunits are demonstrated on the surface of one receptor and the ionophore is seen in cross-section on the other receptor. On stimulation of the two α subunits by two molecules of acetylcholine, the ionophore opens to allow the passage of the end-plate current. (Reproduced with kind permission of Professor WC Bowman.)
Activation of the receptor requires both α sites to be occupied, producing a structural change in the receptor complex that opens the central channel running between the receptors for a very short period, about 1 ms (Fig. 6.2). This allows movement of cations such as Na+, K+, Ca2 + and Mg2 + along their concentration gradients. The main change is influx of Na+ ions, the end-plate current, followed by efflux of K+ ions. The summation of this current through a large number of receptor channels lowers the transmembrane potential of the end-plate region sufficiently to depolarize it and generate a muscle action potential sufficient to allow muscle contraction.
Acetylcholine receptors are also present on the presynaptic area of the nerve terminal. These are of a slightly different structure to the postsynaptic nicotinic receptors (α3β2). It is thought that a positive feedback mechanism exists for the further release of acetylcholine, such that some of the released molecules of acetylcholine stimulate these presynaptic receptors, producing further mobilization of the neurotransmitter to the readily releasable sites, ready for the arrival of the next nerve stimulus (Fig. 6.3). Acetylcholine activates sodium channels on the prejunctional nerve membrane, which in turn activate voltage-dependent calcium channels (P-type fast channels) on the motor neurone causing an influx of calcium into the nerve cytoplasm to promote further acetylcholine release.
FIGURE 6.3 Acetylcholine receptors are present on the shoulders of the axon terminal, as well as on the postjunctional membrane. Stimulation of the prejunctional receptors mobilizes (MOB) the vesicles of acetylcholine to move into the active zone, ready for release on arrival of another nerve impulse. The mechanism requires Ca2 + ions. (Reproduced with kind permission of Professor WC Bowman.)
PHARMACOLOGY OF NEUROMUSCULAR TRANSMISSION
Depolarizing Neuromuscular Blocking Agents
Succinylcholine Chloride (Suxamethonium)
This quaternary ammonium compound is comparable to two molecules of acetylcholine linked together (Fig. 6.4). The two quaternary ammonium radicals, N+(CH3)3 have the capacity to cling to each of the α units of the postsynaptic acetylcholine receptor, altering its structural conformation and opening the ion channel, but for a longer period than does a molecule of acetylcholine. Administration of succinylcholine therefore results in an initial depolarization and muscle contraction, termed fasciculation. As this effect persists, however, further action potentials cannot pass down the ion channels and the muscle becomes flaccid; repolarization does not occur.
FIGURE 6.4 The chemical structures of acetylcholine and succinylcholine. The similarity between the structure of succinylcholine and two molecules of acetylcholine can be seen. The structure of decamethonium is also shown. The quaternary ammonium radicals N+(CH3)3 cling to the α subunits of the postsynaptic receptor.
Inherited Factors: The exact structure of plasma cholinesterase is determined genetically, by autosomal genes, and this has been completely defined. Several abnormalities in the amino acid sequence of the normal enzyme, usually designated , are recognized. The most common is produced by the atypical gene,
, which occurs in about 4% of the Caucasian population. Thus a patient who is a heterozygote for the atypical gene
demonstrates a longer effect from a standard dose of succinylcholine (about 30 min). If the individual is a homozygote for the atypical gene
, the duration of action of succinylcholine may exceed 2 h. Other, rarer, abnormalities in the structure of plasma cholinesterase are also recognized, e.g. the fluoride
and silent
genes. The latter has very little capacity to metabolize succinylcholine and thus neuromuscular block in the homozygous state
lasts for at least 3 h. In such patients, non-specific esterases gradually clear the drug from plasma.
keep the patient anaesthetized and the lungs ventilated artificially, and
monitor neuromuscular transmission accurately, until full recovery from residual neuromuscular block.
Acquired Factors: In these instances, the structure of plasma cholinesterase is normal but its activity is reduced. Thus, neuromuscular block is prolonged by only minutes rather than hours. Causes of reduced plasma cholinesterase activity include:
liver disease, because of reduced enzyme synthesis.
carcinomatosis and starvation, also because of reduced enzyme synthesis.
pregnancy, for two reasons: an increased circulating volume (dilutional effect) and decreased enzyme synthesis.
anticholinesterases, including those used to reverse residual neuromuscular block after a non-depolarizing muscle relaxant (e.g. neostigmine or edrophonium); these drugs inhibit plasma cholinesterase in addition to acetylcholinesterase. The organophosphorus compound ecothiopate, once used topically as a miotic in ophthalmology, is also an anticholinesterase.
other drugs which are metabolized by plasma cholinesterase, and which therefore decrease its availability, include etomidate, propanidid, ester local anaesthetics, anti-cancer drugs such as methotrexate, monoamine oxidase inhibitors and esmolol (the short-acting β-blocker).
cardiopulmonary bypass, plasmapheresis.
Side-Effects of Succinylcholine
Muscle Pains: These occur especially in the patient who is ambulant soon after surgery, such as the day-case patient. The pains, thought possibly to be caused by the initial fasciculations, are more common in young, healthy patients with a large muscle mass. They occur in unusual sites, such as the diaphragm and between the scapulae, and are not relieved easily by conventional analgesics. The incidence and severity may be reduced by the use of a small dose of a non-depolarizing muscle relaxant given immediately before administration of succinylcholine, e.g. gallamine 10 mg (which is thought to be most efficacious in this respect) or atracurium 2.5 mg. However, this technique, termed pre-curarization or pretreatment, reduces the potency of succinylcholine, necessitating administration of a larger dose to produce the same effect. Many other drugs have been used in an attempt to reduce the muscle pains, including lidocaine, calcium, magnesium and repeated doses of thiopental, but none is completely reliable.
Increased Intraocular Pressure: This is thought to be caused partly by the initial contraction of the external ocular muscles and contracture of the internal ocular muscles after administration of succinylcholine. It is not reduced by pre-curarization. The effect lasts for as long as the neuromuscular block and concern has been expressed that it may be sufficient to cause expulsion of the vitreal contents in the patient with an open eye injury. This is unlikely. Protection of the airway from gastric contents must take priority in the patient with a full stomach in addition to an eye injury, as inhalation of gastric contents may threaten life.
Increased Intragastric Pressure: In the presence of a normal lower oesophageal sphincter, the increase in intragastric pressure produced by succinylcholine should be insufficient to produce regurgitation of gastric contents. However, in the patient with incompetence of this sphincter from, for example, hiatus hernia, regurgitation may occur.
Hyperkalaemia: It has long been recognized that administration of succinylcholine during halothane anaesthesia increases the serum potassium concentration by 0.5 mmol L–1. This effect is thought to be caused by muscle fasciculation. It is probable that the effect is less marked with the newer potent inhalational agents, e.g. isoflurane, sevoflurane. A similar increase occurs in patients with renal failure, but as these patients may already have an elevated serum potassium concentration, such an increase may precipitate cardiac irregularities and even cardiac arrest.
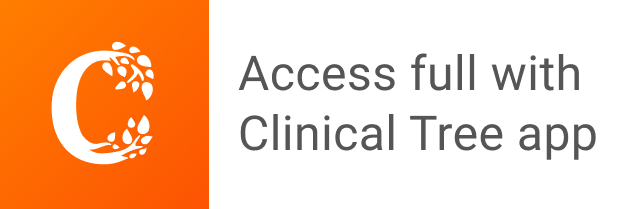