Abstract
In this era of technology, available monitoring devices in the operating room are multiplying and becoming less and less invasive. They provide insight into multiple systems physiology and help achieving the maintenance of homeostasis, which is of particular importance during neuroanesthesia procedures to avoid secondary insult to an already suffering central nervous system. We here review all monitors that are of potential interest during neurosurgical procedures, with special emphasis on their clinical utility and the interest of multimodality monitoring. The monitors that are routinely used during all anesthetic procedures are left apart. We start with temperature, and go on with the monitors that allow checking for adequate oxygen delivery to the brain, adequate brain hemodynamics, and metabolism, including arterial blood pressure, cardiac output, intravascular volume status, hemoglobin concentration, jugular venous oxygen saturation, near infrared spectroscopy, and transcranial Doppler ultrasonography. Intracranial pressure monitoring follows, as well as electroencephalography and depth of anesthesia monitoring. We finish with a few words on decision-helping systems and with some interesting clinical trick in the monitoring domain.
Keywords
Homeostasis, Intraoperative, Monitoring, Multimodal, Neuroanesthesia
Outline
Introduction 161
Temperature 162
Oxygen Transport, Hemodynamics, and Brain Metabolism 162
Arterial Blood Pressure 162
Cardiac Output 163
Intravascular Volume Status 164
Hemoglobin Concentration 164
Jugular Venous Oxygen Saturation 166
Near Infrared Spectroscopy 166
Intraoperative Indications of Near Infrared Spectroscopy 166
Limitations of Near Infrared Spectroscopy 168
Transcranial Doppler Ultrasonography 168
Intracranial Pressure Monitoring 171
Electroencephalography and Depth of Anesthesia Monitoring 173
Electroencephalography, Electrocorticography, and Evoked Potentials for Monitoring Nervous System Integrity 173
Hypnotic Component of Anesthesia Monitoring 173
Nociception Monitoring 174
Miscellaneous 174
Integration of Information and Decision-Helping Systems 175
Clinical Pearls 176
Inaccessible Forehead for Electroencephalographic Monitoring 176
References 176
Introduction
The intraoperative anesthetic management of neurosurgical patients, particularly in case of intracranial neurosurgery, necessitates close attention to maintaining perfect homeostasis, to avoid secondary lesions to eventually suffering neural tissues. This involves rapid and smooth anesthetic depth transitions, adequate hypnotic and antinociceptive depth, hemodynamic stability, minimal interference with cerebral circulation, control of intracranial pressure (ICP) and cerebral perfusion pressure (CPP), temperature, hematosis, and, ideally, neuroprotection. Specific situations also require avoidance of a too marked interference with recordings that aim at assessing nervous system integrity. In addition to classical anesthesia monitoring, including electrocardiography, pulse oximetry, noninvasive blood pressure, and respiratory gas concentration, flow and pressure monitoring, several dedicated monitors may be of utility in achieving these specific goals of neuroanesthesia and eventually help improving patient outcome. They will be reviewed in this chapter, with emphasis on their basic principles, their routine use, the additive value of multimodality, and their demonstrated clinical benefits.
Temperature
Temperature control during neuroanesthetic procedures is mandatory. Hyperthermia is known to worsen computed tomography–evidenced lesions and the clinical status of brain-injured patients, and hypothermia is associated with undesirable side effects including alterations of pharmacokinetics and pharmacodynamics of anesthetic agents, shivering, discomfort upon recovery, sympathetic nervous system activation, increase of myocardial workload, arrhythmias, coagulation disorders, facilitated wound infection, delayed healing, and immune deficit. Active warming and tight temperature control lower the occurrence of hypothermia-related adverse events. Hence, maintenance of normothermia is the rule for most neurosurgical procedures and is a requirement for a good quality early recovery after intracranial neurosurgery and adequate subsequent neurological follow-up. Apart from its deleterious effects, hypothermia has been advocated as a potential intraoperative neuroprotective measure in specific neurosurgical procedures but has never proved to be beneficial in terms of outcome, except for deep hypothermia during the rarely occurring surgical treatment of giant aneurism involving prolonged circulatory arrest.
Temperature can be reliably measured using either thermistors or thermocouples. Thermistors are composed of a semiconductor, whose impedance increases with temperature. Thermocouples contain two metals, and the electric potential difference between them changes with temperature. Other devices are based on infrared emission and measure temperature in the external auditory canal, or they contain a liquid crystal sensor that can be applied on the skin, but they are less frequently used in the operating room.
Temperatures of interest during neurosurgical procedures are mainly core and brain temperatures, which are not necessarily identical. Routinely monitored sites generally allow obtaining only estimates of those temperatures. Tympanic temperature is probably the closest to brain temperature, but the risk of tympanic lesion by the probe is not negligible. Gold standard assessment of core temperature is the central blood temperature measured at the level of a pulmonary artery catheter. However, those catheters are rarely necessary for hemodynamic control during anesthesia for neurosurgery. Reliable alternatives for measuring core temperature are esophageal, bladder, and rectal temperatures. Temperature monitoring and active control for all intracranial procedures and procedures that last for more than 1 h sound reasonable.
Oxygen Transport, Hemodynamics, and Brain Metabolism
To fulfill metabolism requirements, adequate oxygen delivery to all parts of the central nervous system must be constantly insured. This requires effective hematosis in the lungs, high enough blood hemoglobin concentration, and adequate cardiac output. Aside from global systemic transport, oxygen delivery to the brain also depends on global and regional cerebral hemodynamics, which is under the dependency of physiological regulating systems, and may be impeded by pathological processes, iatrogenic interventions, or by medications. Raised ICP and decreased CPP, vasospasms, or hypocarbia are examples of such. Several monitoring devices, either invasive or noninvasive, may be used to control for the adequacy of all intervening elements.
Arterial Blood Pressure
Intermittent noninvasive blood pressure monitoring is standard during all anesthetic procedures and is considered to be enough control for most moderately invasive spine surgeries. A beat-to-beat control of blood pressure is necessary when managing fragile patients or during potentially bleeding surgeries, as well as during all intracranial procedures. Indeed, such a tight control and invasive monitoring allows maintaining adequate cerebral perfusion and limiting the risk of bleeding, either during the course of the procedure or upon recovery. Invasive arterial blood pressure monitoring may also be of help when assessing the intravascular volume status and for drawing arterial blood samples and performing gas analysis.
The entire set for invasive blood pressure monitoring includes an arterial cannula, a continuous irrigation system through infusion of saline under pressure, a pressure transducer that transforms the mechanical signal into an electrical one, and a monitor with a display screen. Zeroing is required at starting of use, with the transducer placed at the horizontal level of the heart. Site for arterial cannula insertion may vary, the most frequent being the radial artery at the wrist. Ulnar, pedal, and femoral arteries can also be used. Humeral artery should be avoided because its distal territory cannot be supplied by collateral vascularization. Strict sterile conditions must be respected during insertion, and echo guiding may be of help for difficult cases ( Fig. 9.1 ).

Continuous beat-to-beat measurement of blood pressure is also possible noninvasively, using volume clamp or arterial tonometry methods. The volume clamp derives blood pressure according to pressure variations in a finger cuff, so as to keep finger artery volume constant. Arterial tonometry uses fingertip plethysmography and pulse volume amplitude. Some authors have evaluated the use of such systems during neurosurgical procedures, in patients where arterial blood sampling is not expected to be necessary. The idea was to avoid the well-known complications of an arterial cannula, including vessel or nerve trauma, pseudo-aneurysm formation, bleeding due to unnoticed disconnection, local hematoma, infection, arterial thrombosis, distal embolism, and ischemia. Although some studies have reported acceptable bias as compared to invasive intra-arterial measurement, a recent meta-analysis has warned about out of acceptable range inaccuracy and imprecision.
Blood pressure management during a neurosurgical procedure must take account of patient comorbidities, risk of bleeding, and brain perfusion. Large hemodynamic variations frequently occur during induction of and recovery from general anesthesia, and practitioners should seek after stability as much as possible. However, perfusion pressure is not the only determinant of cerebral blood flow. In addition to CPP, autonomic regulation, metabolism coupling, and cerebrovascular reactivity to CO 2 and O 2 , acute or chronic changes in global cardiac output play a role. Cerebral blood flow autoregulation should be regarded as a dynamic integrated process, displacing the lower limit, upper limit, and plateau height of the autoregulatory perfusion pressure curve as a function of circumstances. Hence, combining blood pressure monitoring with estimates of cardiac output and cerebral circulation seems to be sound and might reveal efficient at ameliorating patient outcome in the future.
The respiratory variation of the blood pressure curve also offers simple means for assessing the intravascular volume status. This point is developed later in the chapter.
Cardiac Output
Assessment of cardiac output during intracranial neurosurgery has recently been advocated as a potential interesting monitoring to help optimizing cerebral hemodynamics. However, systematic cardiac output monitoring in a neurosurgical context is not the rule, except for very specific and rare procedures such as giant aneurysm clipping under circulatory arrest and in patients with severe cardiovascular comorbidities.
Gold standard methods of cardiac output measurement are the classical thermodilution techniques through a pulmonary artery catheter and transthoracic or transesophageal echocardiography. For neurosurgical procedures, and in otherwise healthy patients, transesophageal echocardiography is mainly used during surgery in the sitting position in case of patent oval foramen to detect eventual paradoxical air embolism. Transesophageal Doppler of the aorta can also estimate the stroke volume from the aortic velocity waveform and is sometimes considered as a reference measure of cardiac output. Its accuracy highly depends on probe placement and on any modification of the aortic diameter such as in case of intra-aortic balloon or coarctation. Several noninvasive devices are also proposed with variable performance, accuracy, and precision. Electrical thoracic bioimpedance relies on the assumption that thoracic impedance changes parallel to those of stroke volume. The limitations of the technique include electrode positioning, electrical interference, abnormal fluid inside the thorax, changes in peripheral vascular resistance, age, gender, arrhythmias, and movement. Thoracic bioreactance is a variant, which takes account of thoracic voltage phase shift and has almost the same limitations, except that it can still provide an estimate of cardiac output in case of cardiac arrhythmia because it averages values over several seconds. Cardiac output may also be derived from the arterial pressure contour, obtained either directly or from the noninvasive methods described above, and using variable algorithms. All these techniques are highly dependent on arterial pressure waveform signal quality, and therefore on eventual finger edema, intense vasoconstriction, sensor position, and movement. Some of them necessitate calibration and others not.
The clinical utility and reliability of cardiac output measurement in neuroanesthesia have been relatively poorly studied. Thoracic bioimpedance has been demonstrated to reliably mirror the hemodynamic changes associated with crucial events during neurosurgical procedures such as induction, scalp infiltration, changes in anesthetic depth, or mannitol administration. Stroke volume monitoring is also sometimes used to guide fluid administration during intracranial neurosurgery. Up to now, scientific evidence does not support equivalence between invasive and noninvasive methods of hemodynamic monitoring and the lasts cannot replace the formers when close monitoring is needed, such as during surgery in the sitting position.
Intravascular Volume Status
Substantial fluid shifts can occur during neurosurgical procedures, either as a consequence of blood loss or following the administration of medications aiming at improving brain relaxation, such as mannitol or furosemide. Hence, controlling for the intravascular volume status is essential. Several methods are available of which some are simple and easy to implement. Indeed, central venous pressure and pulmonary capillary wedge pressure are no longer considered as gold standards, at least in patients under positive pressure ventilation.
The invasive arterial pressure waveform allows measuring the delta pulse pressure (DPP) or the delta-down (DD) intermittently ( Fig. 9.2 ). To do so, the patient must be in cardiac sinus rhythm and under mechanical ventilation. The tidal volume should be set at least at 8 mL/kg, and the frequency rate reduced to values as low as 4 per min to mimic respiratory pauses. DPP corresponds to the difference between the maximal and minimal pulse pressure during one breathing cycle, divided by the mean of those two pulse pressures, while DD is the difference between the systolic arterial pressure at the end of a 5-s respiratory pause, immediately before lung inflation, and its minimal value during the course of one respiratory cycle. Values of DPP higher than 13%, and values of DD higher than 5 mmHg are indicative of an increased probability of preload cardiac output dependency and a positive hemodynamic response to fluid loading. Both indices have been demonstrated to be accurate in the context of intracranial neurosurgery. Their main disadvantages are their intermittent nature and susceptibility to confounding factors, including nonsinus cardiac rhythm, ventilation volume and pressure, vasopressor administration, abdominal pressure, and cardiac failure. Dynamic parameters may have additive value, insofar as they offer a continuous assessment of the intravascular volume. They are based on systolic pressure or stroke volume variability as assessed by pulse contour analysis of an arterial or plethysmographic waveform. Using such technology, several commercially available devices provide indexes of susceptibility to fluid loading. Closed-loop systems of fluid administration have even been proposed. Accumulating evidence suggests that goal-directed fluid administration is beneficial for enhanced recovery after specific types of surgeries, but this question has still not been fully addressed for neurosurgical procedures.

Hemoglobin Concentration
The main oxygen transporter in the blood is hemoglobin. During potentially bleeding neurosurgical procedures and in patients with preexisting anemia, it is necessary to have prompt access to rapid, accurate, and reliable hemoglobin concentration measuring devices. Aside from automated laboratory hemoglobin measurement systems, which may be too time demanding particularly in the context of an emergency, several point-of-care devices are proposed.
Blood sample–requiring systems at the bedside use reagent-based or nonreagent-based absorption photometry at multiple wavelengths or conductivity. Other noninvasive, and hence non–blood sample–requiring devices involve multiple wavelength co-oximetry, occlusion spectroscopy, or transcutaneous reflection spectroscopy technologies. Several studies have been performed to compare the results obtained with those devices and those obtained using automated laboratory systems or between them ( Table 9.1 ), not necessarily in the context of neurosurgery. Although bias as compared to laboratory values is generally small, the limits of agreement may be large with a significant amount of outliers. In addition, discrepancies may vary according to real hemoglobin level and several confounding factors. Pulse co-oximetry may be confounded by ambient light, bilirubin, hemoglobin variants, intravascular dyes, motion, sensor positioning, temperature, venous congestion, edema, high tissue lipid content, and hypovolemia. Although having been demonstrated to be indicative of hemoglobin level during complex spine surgery, pulse co-oximetry remains less accurate than conventional laboratory measurements. Accuracy may improve when calibrating with a baseline laboratory testing at the beginning of the procedure, particularly in children undergoing neurosurgery. Those noninvasive monitors provide more timely information, but should be considered as trend monitors rather than absolute indicators of transfusion need.
Commercial Devices | Technology | Blood Sample Needed | Comparison With Automated Laboratory Measurements MD (LA) |
---|---|---|---|
Hemocue | Reagent-based photometry | Yes | 0.08 (−1.3–1.4) |
Diaspect | Nonreagent-based photometry | Yes | −0.18 (−2.05–1.68) |
i-Stat | Conductivity | Yes | 0.05 (−1.16–1.25) |
CO-Oxi | Multiple wavelength co-oximetry | No | −0.03 (−3.0–2.9) |
OrSense | Occlusion spectroscopy | No | −0.66 (−3.39–2.09) |
Haemospect | Transcutaneous reflection spectroscopy | No | −0.22 (−2.64–2.21) |
Jugular Venous Oxygen Saturation
Near infrared spectroscopy (NIRS) combined with transcranial Doppler ultrasonography (TCDU) and jugular venous oxygen saturation (SjvO 2 ) monitoring are means for appreciating brain hemodynamics and oxygen delivery. SjvO 2 monitoring requires the retrograde placement of a catheter into a jugular vein, up to the jugular bulb, to monitor the mixed cerebral venous blood and above the C1/C2 level to limit contamination by the facial venous blood. Side for placement should preferably be the dominant side of the brain or the side with the most prominent pathology, if any. Oxygen saturation may be measured in regularly drawn blood samples or using a fiber-optic catheter and light absorption in the red/infrared wavelength range. Although generally quite easily placed, the catheter may be the source of rare complications, including hematoma, infection, thrombosis, raised ICP, or incidental wrong vessel puncture.
SjvO 2 reflects the balance between oxygen delivery to the brain and cerebral metabolic consumption of oxygen. Normal values range between 50% and 75%. Decreases in SjvO 2 can be seen in case of systemic or local oxygen supply deficiency, including hypoxia, low blood pressure, decreased CPP, embolism, or vasospasm ( Table 9.2 ), and in case of increased oxygen consumption including hyperthermia and seizure. Causes of SjvO 2 values above normal range can be classified into those related to a decrease in cerebral metabolism, restricted oxygen diffusion or extraction, shunting, increased oxygen supply, or a combination of these. For example, a global decrease in cerebral metabolism can be observed during hypothermia. Infarction or inflammation causes restriction in oxygen diffusion and extraction, as well as microvascular shunting. Increased oxygen supply is observed during hyperoxia, and hypercarbia can be responsible for substantial shunting.
Low SjvO 2 (<50%) | ||||||||
---|---|---|---|---|---|---|---|---|
Systemic Oxygen Supply Deficiency | Local Oxygen Supply Deficiency | Increased Cerebral Oxygen Consumption | ||||||
Cause | Diagnosis | Correction | Cause | Diagnosis | Correction | Cause | Diagnosis | Correction |
Hypoxia |
|
| Embolism Thrombosis |
|
| Hyperthermia |
|
|
↓ CPP
|
|
| Vasospasm |
|
| Seizure |
|
|
Anemia |
|
| ||||||
Low CO |
|
|
High SjvO 2 ( > 75%) | ||||||||
---|---|---|---|---|---|---|---|---|
Decrease in Cerebral Metabolism | Restricted Oxygen Diffusion | Restricted Oxygen Extraction | ||||||
Cause | Diagnosis | Correction | Cause | Diagnosis | Correction | Cause | Diagnosis | Correction |
Hypothermia |
|
| Infarction |
|
| Infarction | Same as restricted oxygen diffusion | |
Deep sedation |
|
| Inflammation |
|
| Inflammation |
Microvascular Shunting | Shunting | Increase in Oxygen Supply | ||||||
---|---|---|---|---|---|---|---|---|
Cause | Diagnosis | Correction | Cause | Diagnosis | Correction | Cause | Diagnosis | Correction |
|
|
|
|
|
|
|
|
Interpretation of SjvO 2 values may be uneasy in some instances. It is a global measure and it may miss eventually occurring focal brain ischemia. When brain tissue death is massive, SjvO 2 can be high as a consequence of shunting. Classical causes of wrong SjvO 2 values are displacement of the catheter, thrombus around its tip, and contamination by facial venous blood. Anesthesia may also have an influence on SjvO 2 values. Transient desaturations have been reported under propofol anesthesia, more frequently in normothermic than in hypothermic patients, while SjvO2 increases have been observed under sevoflurane anesthesia. This is due to the different properties of those agents on cerebral vasculature, propofol being vasoconstrictive, and sevoflurane vasodilating. Side of recording may also be important, insofar as cerebral venous drainage usually predominate on one side through the dominant jugular bulb.
SjvO 2 has mostly been used to guide therapy in the intensive care unit for traumatic brain injury and subarachnoid hemorrhage patients. However, its intraoperative use during intracranial neurosurgery allows commonly detecting desaturation episodes, and its combination with TCDU may impact on clinical decision-making to optimize cerebral physiology. Large randomized clinical trials investigating the impact of intraoperative SjvO 2 monitoring on patient outcome are lacking.
Near Infrared Spectroscopy
NIRS consists in measuring near infrared light absorption by the superficial part of brain tissues. Light is emitted through the skin and the skull, entering the brain tissue to a depth of approximately 1–2 cm. Absorption at different wavelengths allows estimating the mixed capillary–venous regional cerebral oxygen saturation (rScO 2 ). rScO2 depends on the balance between local metabolism and oxygen delivery, which in turn depends on regional cerebral blood flow and blood oxygen content. If metabolism is constant, rScO 2 can be regarded as a surrogate measure of regional cerebral blood flow changes. The system necessitates the use of a noninvasive scalp probe and allows continuous monitoring of rScO 2 over a restricted area of approximately 1 cm 2 .
Normal rScO 2 values at atmospheric oxygen levels range from 62% ± 6% in cardiac surgery patients to 71% ± 6% in healthy young men. Low preoperative baseline rScO 2 values are predictive of increased 30-day morbidity and mortality. There is no consensus in the literature on the rScO 2 threshold value indicating significant desaturation, but a decline of more than 20% as compared to baseline is generally accepted as a predictor of cerebral ischemia. It is also generally agreed that both depth and duration of desaturation are important. A less extreme threshold of 10% should probably prompt therapeutic intervention to raise rScO 2 .
Intraoperative Indications of Near Infrared Spectroscopy
At the extremes of age, in the very young and in the elderly over 60 years, the brain is at risk of injury during surgery and anesthesia. In addition to the sensitivity of their brain to the effects of anesthetic agents, aged patients are prone to experience postoperative cognitive decline and develop delirium or stroke. Evidence accumulates demonstrating that cerebral oxygen deprivation episodes lead to adverse clinical outcomes including cognitive decline, major organ dysfunction, and prolonged hospital length of stay, both in cardiac and noncardiac surgery patients.
The intraoperative use of NIRS was initially described for aortic arch surgeries and carotid endarterectomies. Despite an increasingly large use of rScO 2 monitoring during cardiac surgery, Zheng et al. were not able, in their systematic review, to strongly link cerebral desaturations, and interventions to improve it, with the incidence of postoperative neurological complications. Indeed, most of NIRS observational studies in aortic arch surgery patients have small sample sizes, and a very low incidence of strokes, rendering the assessment of the relationship between cerebral desaturation and neurological outcome uneasy. Some case reports, as well as observational studies, have shown the usefulness of NIRS to warn about cannula malposition and failure of cardiopulmonary bypass. During carotid endarterectomy, the most efficient approach to cure reduced rScO 2 is the insertion of a shunt. NIRS has a sensitivity of approximately 75% and a specificity of 98% at correctly indicating the need for shunting. After clamp release, an increase in rScO 2 of more than 20% predicts the occurrence of a cerebral hyper-perfusion syndrome. Regarding nonvascular surgical procedures, a review performed by Nielsen identified surgeries where a decrease in rScO 2 is reported. These surgeries include procedures in the Fowler position, which are characteristic of shoulder and laparoscopic surgeries, for example. Hip surgery and single lung ventilation thoracic surgery are also at risk of decrease in rScO 2 .
The link between repeated occurrences of cerebral desaturation and worse postoperative outcome is, however, not straightforward. A pronounced intraoperative cerebral desaturation does not necessarily lead to postoperative cognitive decline after shoulder surgery in the beach chair position. In addition, an association between cerebral desaturation and outcome parameters such as postoperative wound infection, kidney failure, and myocardial infarction remains to be established. Current evidence points toward worse outcome, including postoperative cognitive decline, kidney dysfunction, and prolonged hospital length of stay in cardiac surgery patients having experienced severe cerebral desaturation, as well as in patients having been submitted to certain types of other noncardiac surgeries. Even if more studies are needed to demonstrate a clear association, the overall risk to benefit ratio goes in favor of the intraoperative utility of NIRS, all the more since it is noninvasive, continuous, and has a moderate cost.
Limitations of Near Infrared Spectroscopy
Several confounding factors may impede the adequate interpretation of rScO2 values. A contamination by blood originating from the external carotid artery territory may occur, and the extent to which this contamination may skew NIRS accuracy varies from a device to another. External carotid contamination may be as high as 20%. An influence of anatomic variability in skull shape, for example enlarged frontal sinus, or uncommon cerebral venous drainage through superior sagittal veins must be considered. When vasopressors such as noradrenaline are administered to maintain arterial pressure, up to one-third of changes in rScO2 can be accounted for by changes in skin blood flow. Ephedrine, by increasing mean arterial pressure without affecting cardiac output, does not change rScO2, while pure α 1 -adrenergic agonists such as phenylephrine do. In that case, the decrease in rScO2 is secondary to a drop in cardiac output. It may therefore be advised to check for an adequate intravascular volume status before interpreting changes in rScO2 following the administration of a vasopressor. In patients with a liver disease, high bilirubin plasma concentrations alter NIRS measurements through the competitive absorption of light by this pigment. Another limiting factor is the limited frontal brain area where NIRS can measure oxygenation. Embolism or ischemia occurring in distant areas can therefore be undetected . Finally, commercial cerebral oximetry devices assume a fixed value of either 70:30 or 75:25 for the venous to arterial blood volume ratio. Relative changes in the volume of one compartment or the other will change the overall cerebral hemoglobin saturation, without any real change in the saturation of each individual compartment.
Transcranial Doppler Ultrasonography
In 1982, Aaslid was the first to describe the use of transcranial Doppler for measuring cerebral blood flow velocity. Ultrasound brain exploration has long been limited to Doppler exploration, but technological advances now allow grossly imaging the main brain structures using echography. Through the visual recognition of the insonated vessel, it has considerably facilitated Doppler use and has added morphological elements that can be useful to diagnosis. TCDU is the only noninvasive and cost-effective bedside tool that provides real-time information on cerebral hemodynamics. It has no contraindications, and its technical limitations can often be overcome by the intravenous administration of a sonography contrast agent. In adults, insonation usually occurs through the four commonly defined acoustic windows, including the temporal, orbital, suboccipital, and submandibular window. These windows are characterized by a thinner bone layer, permitting ultrasound penetration.
Ultrasound Basic Physics
Echography necessitates an ultrasound transducer, which acts both as a transmitter and a receiver. Reflection of ultrasound waves occurs at the interface between different tissue types, and depends on the resistance to ultrasound breakthrough, or acoustic impedance. When ultrasounds are reflected, and thus sent back to the transducer, the piezoelectric crystals convert the mechanical energy of the returning echoes into an electrical current, which is processed by the ultrasound machine to produce a two-dimensional gray scale image. During their travel into the tissues, ultrasound waves are attenuated. Attenuation depends on three factors, namely the attenuation coefficient of the tissue, the traveled distance, and the ultrasound frequency. Insofar as bones are substantially impervious to ultrasounds, and that attenuation increases with ultrasound frequency, a low-frequency transducer in the order of 2 MHz is necessary for transcranial ultrasonography.
The flow within vascular structures can be studied using color flow Doppler. Red blood cells moving toward the transducer return waves at a higher frequency than the emitted signal. The corresponding image of flow is displayed in red on the device. Contrarily, when those cells are moving away from the transducer, the returned waves have a lower frequency and are displayed in blue. Color flow Doppler helps identifying arteries through the integration of information coming from the anatomical view, as well as direction and pulsatility of flow. Pulse wave Doppler is based on the Doppler principle described by Christian Doppler in 1843. Exactly like in color flow Doppler, the reflected ultrasound wave has a higher or a lower frequency than the emitted one. This is termed as a positive or negative shift in frequency, respectively. The velocity of red blood cells as measured by a pulse wave Doppler depends on the cosine of the angle of insonation ( Fig. 9.3 ). As a consequence, when the angle of insonation is 0°, the measured velocity equals the true velocity. When the angle is 90°, the measured velocity is 0, irrespective of the actual velocity.

Practical Applications
In the operating room and during neurosurgery, TCDU can be used to seek for hyperemia, vasospasm, microembolic signals, vasomotor reactivity (VMR), and to estimate ICP. These indications are also common in the neurointensive care unit. Other common indications of TCDU are the identification of sickle cell disease children at the highest risk of first-ever stroke, and those in need of blood transfusion. TCDU is also very sensitive and specific to detect right-to-left shunt and to quantify the functional repercussions of such a shunt. The last indication of TCDU is the diagnosis of a cerebral circulatory arrest. This paragraph will be limited to the indications that are of potential utility in the operating room.
Vasospasm and Hyperemia
Vasospasm is a suddenly occurring and rapidly evolving pathology. TCDU is therefore a key tool for its identification and diagnosis, as well as for daily monitoring, appreciation of severity, and circumscription of incriminated territory. A change in mean flow velocity (MFV) often precedes an eventual neurological deficit. Detecting those changes in velocity authorizes to adapt the treatment precociously, and prevent complications.
The threshold for deciding on an increased MFV varies according to the studied artery ( Table 9.3 ). More than an absolute number, the magnitude of a change in mean velocity is also important. An increase of more than 50 cm/s in 24 h is predictive of the occurrence of a vasospasm-related ischemic lesion. In the middle cerebral artery, when the MFV remains between 120 and 200 cm/s, the sensitivity for diagnosing a vasospasm is weak (67%). In that case, only arteriography may provide certainty. Another useful parameter for diagnosing vasospasm in the middle cerebral artery is the Lindegaard’s ratio. It corresponds to the ratio between the MFV in the middle cerebral artery and the MFV in the submandibular internal carotid artery. The Lindegaard’s ratio may also help differentiating hyperemia from vasospasm, which have completely opposite treatments.
Concerned Artery | MCA | MFV | Lindegaard’s ratio for the MCA |
Moderate vasospasm | >120 and <200 cm/s | Hyperemia: <3 Moderate vasospasm: 3–6 Severe vasospasm: >6 | |
Severe vasospasm | >200 cm/s | ||
ACA | MFV | ||
Discreet vasospasm | >80 cm/s | ||
Significant vasospasm | >130 cm/s | ||
PCA | MFV | ||
Discreet vasospasm | >90 cm/s | ||
Significant vasospasm | >110 cm/s | ||
Vertebral artery | MFV | ||
Vasospasm | >80 cm/s | ||
Basilar artery | MFV | ||
Vasospasm | >95 cm/s |
Microembolic Signals or High Intensity Transient Signals
During carotid surgery, monitoring an intracranial artery distal to the steno-occlusive site allows detecting spontaneous embolic signals and quantifying the embolic potential of the atherosclerotic plaque. According to the International Cerebral Hemodynamics Society, those signals are characterized by a high intensity, that is more than 10 dB higher than blood flow, a random occurrence during the cardiac cycle, a unidirectional nature, a short duration (10–100 ms), and an audible component (explosive signal) ( Fig. 9.4 ). This kind of surveillance is useful not only during carotid surgery, but also during aortic, cardiac, or even orthopedic surgery. It helps identifying the critical phase of surgery, when the risk of embolism is important. Detecting such a high risk should prompt corrective measures at the level of the equipment (e.g., extracorporeal circulation) or the surgical technique.

Vasomotor Reactivity
VMR represents the ability of cerebral vessels to maintain a near constant blood flow in response to a vasomotor stimulus. TCDU allows a real-time observation of vasomotor changes in response to various stimuli. Concerned stimuli can be hemodynamic stimuli such as a rapid leg cuff deflation or a Valsalva maneuver or the transient hyperemia response to medications such as acetazolamide, breath-holding, and CO 2 inhalation. CO 2 is the strongest vasodilation stimulus to the cerebral circulation. Several factors may influence VMR, including gender and level of estrogenic impregnation, as well as chronic cardiovascular pathologies such as chronic high blood pressure and diabetes. In those two last cases, VMR is reduced.
A simple method for measuring VMR has been described by Markus et al. It consists in calculating the breath-holding index (BHI) as follows:
MFVend − MFVbaseline MFVbaseline × 100 seconds of breath holdings
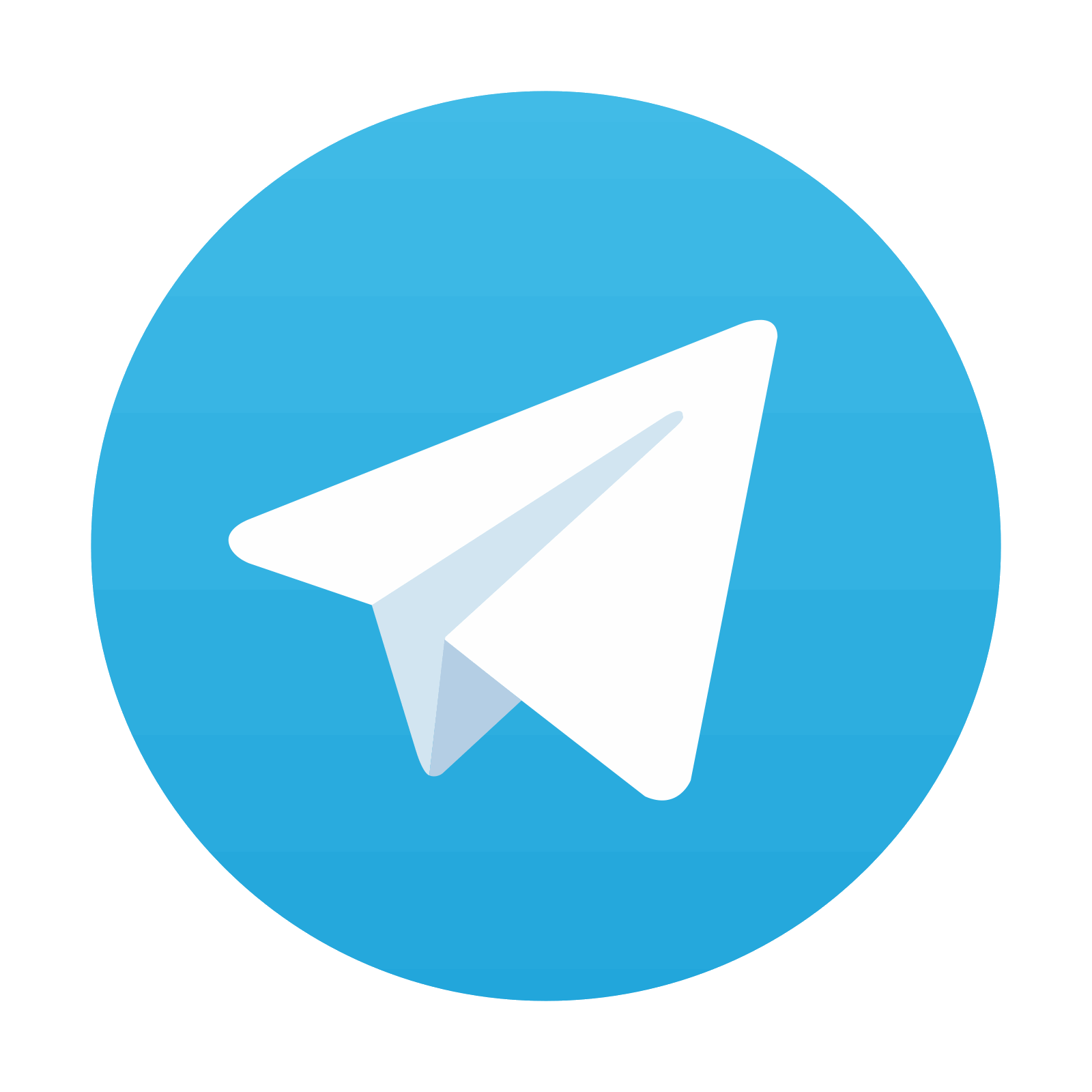
Stay updated, free articles. Join our Telegram channel
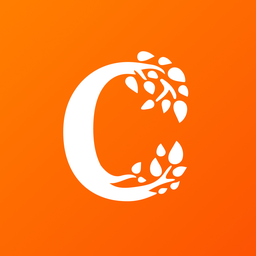
Full access? Get Clinical Tree
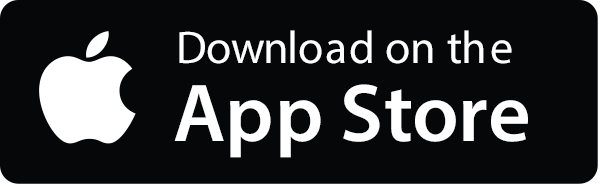
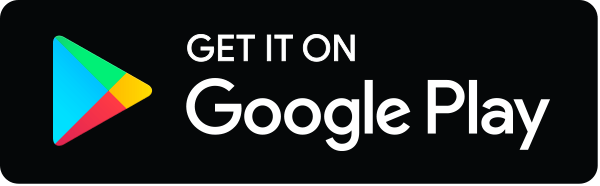