Organ system
Optical signals
Electrical signals
Mechanical signals
Acoustic signals
Chemical signals
Central nervous system
NIRS
aEEG
EMG
ABR
SSEP
Respiratory
Gas analysis
Impedance plethysmography
Peak pressure
Tidal volume
Minute ventilation
Acoustic flow sensors
EtCO2
FiO2
Cardiovascular
Pulse oximetry
Hb concentration
Plethysmography index
ECG
Impedance plethysmography
NIBP
Echocardiography
Before starting any surgical procedure, it is necessary to determine which organ systems are at risk of injury, the degree of risk, the manifestation of injury, and the impact of injury on the outcome of the operative procedure. This process guides the selection of specific systems to be monitored and the type of monitor to be used. Monitoring is a cognitive exercise in integrating and interpreting information from multiple sources to assign a value of “normal” or “abnormal” to the present functional state and to predict outcome.
The Respiratory System
Neonates have less ability to compensate for impairment in respiratory function than adults. Consequently, continuous monitoring of the respiratory system in neonates during surgery and anesthesia is particularly critical. The main devices to monitor the respiratory system include pulse oximetry, oxygen and carbon dioxide gas analyzers, as well as ventilatory pressure, volume, and rate (apnea) monitors.
Pulse Oximetry:
The use of pulse oximetry to measure arterial oxygen saturation in anesthesiology is standard of care. Pulse oximetry relies on the Beer–Lambert law, which relates the concentration of a chromophore to light absorption, optical path length, and extinction coefficient. In pulse oximetry, the chromophores of interest are oxy and deoxyhemoglobin. Because there are two chromophores, two wavelengths in which the extinction coefficient of oxy and deoxyhemoglobin differ are required to determine oxygen saturation. These are 660 and 940 nm. Neonatal hemoglobin and adult hemoglobin have identical absorption spectrum, and thus pulse oximetry is equally accurate in neonates as in adults. The pulse oximeter calculates oxygen saturation as the ratio of absorbance of the pulsatile optical signal and the non-pulsatile optical signal at two wavelengths, represented by
where AC is the pulsatile signal and DC is the non-pulsatile signal. The pulse oximeter converts R to oxygen saturation through an empirically derived curve (Fig. 6.1). Of note, the relationship between R and arterial saturation is curvilinear but closely approximates a straight line over a 20 % range in arterial saturation. The calibration curve is constructed by having healthy adults breathe hypoxic gas mixtures and comparing R to CO-oximeter-measured arterial saturation [1]. This calibration curve originates from arterial saturation of 80–100 %; values less than 80 % are extrapolated from the curve and are less accurate because of the curvilinearity (Fig. 6.1). However, it is possible to construct a calibration curve over a different range than 80–100 %; for example, the Masimo “Blue Sensor” is calibrated for the 60–80 % range for use in congenital heart disease.



Fig. 6.1
Pulse oximeter SpO2 is calculated from the empirical relationship between arterial oxygen saturation and R, the absorbance ratio at red and infrared light
It is important to distinguish fractional oximetry from functional oximetry. Fractional oximetry calculates the fraction of oxyhemoglobin in relation to the four different species of hemoglobin:
Fractional SaO2 is calculated by pulse CO-oximetry (Masimo Corporation) using at least four different wavelengths. On the other hand, functional oximetry is the saturation determined by traditional pulse oximeters that use only two wavelengths:



Pulse oximetry suffers from several limitations. Under conditions of hypothermia, vasoconstriction, hypotension, and motion, pulse oximeters are less accurate and less reliable. Advances in signal processing over the years have improved the accuracy and reliability under these conditions, as does placing the sensor on structures closer to the central circulation (e.g., ear lobe, alar nose, and tongue). Polycythemia and anemia do not affect the accuracy of pulse oximetry. During significant hypoxemia, pulse oximeter measurements exceed the actual saturation, although during acute desaturations, the oximeters usually underestimate the actual saturation. During methemoglobinemia, the SpO2 becomes 85 % regardless of the actual arterial saturation because of the absorbance spectra of methemoglobin relative to hemoglobin. During carbon monoxide exposure, SpO2 overestimates arterial saturation because oxy- and carboxyhemoglobin absorb red light similarly.
In neonates, pulse oximetry is particularly useful to the monitor preductal and postductal arterial saturation, especially in those predisposed to persistent fetal circulation. A decrease in the postductal SpO2 compared with preductal SpO2 of more than 10 % indicates significant pulmonary hypertension and shifts toward fetal circulation. If a right-to-left shunt occurs at the foramen ovale, such a difference would not occur. The preductal and postductal SpO2 during a PDA closure may serve as a guide to avoid erroneous ligation of other great vessels since the PDA can be larger than the aorta and the left pulmonary artery. In some circumstances, maintenance of the transitional circulation is essential to maintain pulmonary and/or systemic blood flow, such as single ventricle lesions, pulmonary atresia, or transposition of great arteries. Monitoring SpO2 estimates the ratio between the pulmonary flow and systemic flow (Qp/Qs) and can serve to gauge the balance of pulmonary blood to systemic blood flow.
Monitoring SpO2 can also track changes in the arterial oxygenation from the fetus to the neonate during the first minutes after birth. During fetal life, normal SpO2 is approximately 72 %. During the initial hours after birth, there is a rapid and progressive increase in SpO2 to the mid-90s [2]. Pulse oximetry has also been evaluated as a screening tool for congenital heart disease in the newborn nursery. If SpO2 does not reach the mid-90s by day two of life, it suggests cyanotic congenital heart disease. Studies have shown pulse oximetry has a specificity of 99 % and a sensitivity of 72 %, superior to a clinical evaluation, to diagnose cyanotic congenital heart disease [3,4]. Oxygen toxicity in preterm infants contributes to development of retinopathy of prematurity, and pulse oximetry is used to maintain SpO2 between 90 and 94 % to limit this risk. Saturations <90 % reduce the risk of retinopathy of prematurity even further but increase the mortality rate and thus are not recommended [5]. Excessive oxygen administration may also be deleterious during resuscitation [6,7]. During neonatal resuscitation, the American Heart Association recommends monitoring the preductal SpO2 to titrate the oxygen administration [8].
Inspired and Expired Gas Analysis:
Measurement of oxygen, carbon dioxide, and anesthetics remains fundamental to anesthesia practice. Initially these concentrations were measured by mass spectrometry but were replaced by infrared spectrophotometry, as CO2, N2O, and inhaled anesthetics have different absorption spectra at 7–13 μm. As with NIRS and pulse oximetry, the concentration of the gases is calculated using the Beer–Lambert law. Oxygen concentration cannot be measured by this technique because it does not absorb infrared light; rather, it is measured by electrochemical or paramagnetic methods.
Expired CO2 concentration or end-tidal CO2 (EtCO2) can be plotted against time to show the changes of CO2 concentration during inspiration and expiration. The continuous measurement of EtCO2 is standard of care in anesthesia practice. Two monitoring systems exist to measure EtCO2: sidestream and mainstream capnography.
Sidestream capnography uses thin tubing connected to the breathing circuit to continuously aspirate gas that flows to a spectrophotometry cell. The flow sampling rates are between 50 and 500 ml/min. In neonates, the high-flow sampling should not be used because it will entrain inspiratory gas leading to an underestimation of the EtCO2 or dramatically decreasing alveolar ventilation if the fresh gas flow is small. Other limitations include water vapor condensation obstructing the tubing, leaks or disconnections at the cell analyzer, and several-second delay from actual to monitored changes in the EtCO2.
Mainstream capnography incorporates the infrared analyzer at the breathing circuit without need for sampling tubing. This results in a more rapid gas analysis. Mainstream capnography offers an advantage in neonates because it eliminates the risks of excessive gas sampling and errors from sampling dead space. However, its disadvantages include the weight of the analyzer kinking the tracheal tube, need for frequent calibration, and water vapor condensation leading to errors and unreliable measurements.
The capnograph provides important clinical information during neonatal anesthesia (Fig. 6.2). The waveform is divided into an inspiratory phase and 3 expiratory phases. The first expiratory phase results from gas in the large airways (anatomical dead space). The second expiratory phase results from the mixed sampling of large airways and alveolar gas (transitional phase). The third expiratory phase results from alveolar gas (plateau phase). During inspiration the EtCO2 rapidly drops to zero. Because of normal physiologic alveolar dead space, a gradient of 1–5 mm Hg normally exists between the PaCO2 and EtCO2. This gradient significantly increases under conditions that increase dead space or decrease cardiac output, which in neonates include right-to-left pulmonary shunt associated with congenital heart disease, meconium aspiration, respiratory distress syndrome, and shock. Also phase III of expiration can change from plateau to an upward slope during conditions of increase alveolar dead space. The PaCO2–EtCO2 gradient should be monitored to gauge pulmonary function. For example, during bronchospasm, the slope of phase III increases from areas of airway obstruction that contain greater CO2 partial pressures and takes more time to be exhaled.


Fig. 6.2
A typical capnogram illustrating the change in carbon dioxide tension during the respiratory cycle
Apnea Monitors:
These monitors are classified based on detection of chest movement (transthoracic impedance), ventilation (capnography), oxygenation (pulse oximetry), or airflow (acoustic). Of the monitors, transthoracic impedance and pulse oximetry are the most popular. Typically, transthoracic impedance is incorporated into ECG monitoring. A small current is transmitted from one ECG pad to another one, and changes in the impedance to this current due to the chest expansion or contraction allow measurement of a respiratory rate. Since transthoracic impedance detects chest movement but not airflow, it misses obstructive apnea, which provides an erroneous respiratory rate. Pulse oximetry is a poor technology to detect apnea because oxygenation changes take time to decrease after the absence of ventilation, especially in neonates who are breathing supplemental oxygen. Capnography as an apnea monitor suffers from unreliability as nasal secretions may obstruct gas sampling to give spurious alarms of apnea. Monitoring the airflow with acoustic sensors located on the neck or chest offers theoretical advantages over transthoracic impedance, capnography, and pulse oximetry. Acoustic apnea monitors sense vibratory sounds produced by turbulent flow in the large airways and converts a sound signal into an electrical signal from which respiratory rate is calculated. Acoustical respiratory rate monitoring has better accuracy and less bias than capnography or impedance technologies [9].
Pulmonary Mechanic Monitors:
Pulmonary mechanics includes inspiratory and expiratory tidal volumes, peak inspiratory pressure, mean airway pressure, positive end-expiratory pressure (PEEP), and inspiratory and expiratory cycle time.
Inspiratory tidal gas volume is defined by the setting on the ventilator. Traditional anesthesia ventilators deliver this predetermined volume which can differ substantially from the actual tidal volume, especially in neonates, because of the compliance of the breathing circuit in relation to pulmonary and chest wall compliance and the fresh gas flows. As a result of its unpredictability, mechanical ventilation in neonates historically used a pressure control mode, which is independent of the breathing circuit compliance and of the fresh gas flows [10,11]. However, during pressure mode ventilation, the actual tidal volume varies according to the total compliance and the airway resistance. Thus, tidal volume may suffer if total compliance decreases or airway resistance increases. Examples of such situations include acute pulmonary edema, insertion of chest or abdominal sponges or retractors, or tracheal tube obstruction.
Advances in the anesthesia ventilators have made the delivery of predetermined tidal volumes during volume control ventilation more predictable. These anesthesia machines monitor compliance in the breathing circuit and compensate for it by adjusting the delivered tidal volume, for example, if fresh gas flows or compliance changes. A common circumstance that changes compliance in the breathing circuit is contraction or expansion of the tubing of the breathing circuit. The anesthesia machine tests for compliance as part of the machine check to compensate appropriately for the different circuit compliance. Thus, the compliance test must be done again if the circuit is changed, contracted, or extended. When the tidal volume of contemporary anesthesia ventilators was set to volume control mode in a model of different lung compliances, they found that volume control ventilation could be used in neonatal anesthesia with state-of-the-art ventilators [12]. However, despite these compensatory mechanisms, modern ventilators are unable to compensate for large circuit leaks regardless of the ventilation control mode, volume, or pressure mode. The use of cuffed tracheal tubes eliminates the main source of circuit leaks in neonates.
During a normal breath, the expired tidal volume is less than the inspired tidal volume because of the greater oxygen uptake at the alveolar–capillary membrane compared with the carbon dioxide output from the capillaries into the alveoli. Measurement of expired tidal volume occurs close to the expiratory valve, which tends to overestimate the actual volume.
Monitoring peak inspiratory pressure and tidal volume in neonates provides information about lung compliance and airway resistance to detect conditions such as an endobronchial intubation, bronchospasm, and tracheal tube obstruction. Monitoring these variables also helps to prevent volutrauma or barotrauma during the ventilator support in the operating room, especially in those neonates with pulmonary disease or immature lungs, in whom small tidal volumes and inspiratory pressures decrease the risk of bronchopulmonary dysplasia, a strategy referred to as permissive hypercapnia. During surgical procedures in which substantial changes in the compliance can occur, the peak inspiratory pressure should be modified accordingly to avoid pressure or volume trauma to the lung parenchyma.
The anesthesia circuit incorporates pneumatic or electronic devices to measure airway pressure (Fig. 6.3). The sensor location varies with the anesthesia workstations, ideally closer to the tracheal tube (Y-piece) to increase its reliability. However, this location increases the dead space, along with risks of disconnections or tracheal kinking. Most frequently, the location is close to the expiratory or inspiratory valves. The device incorporates a diaphragm connected to the breathing circuit which changes in diameter according to the airway pressure, activating a pressure relief valve once the selected peak pressure has been reached. It also detects a leak in the system if a minimal threshold pressure is not reached.


Fig. 6.3
The anesthesia breathing circuit and ventilator and reservoir bag for an anesthesia workstation
The Cardiovascular System
The cardiovascular system delivers substrate to tissue, transports hormones and other message compounds from one organ to another, and removes waste products from the organs. The most easily measured substrate is oxygen, and the most easily measured waste product is carbon dioxide. Quantitative assessment of oxygen delivery requires measurement of cardiac output, oxygen saturation, and hemoglobin concentration. The small size of low- and very-low-birth-weight infants makes use of invasive monitors difficult or impossible and is complicated by the presence of variable shunts which can change over time, making estimates of left ventricular output misleading.
Echocardiography:
Acoustic-based technologies have improved dramatically over the last decade. Echocardiography uses both one- and two-dimensional analysis to generate images and superimpose flow information on the image. Color Doppler translates information about flow direction and velocity into a color map and is useful to assess shunts. Doppler measurements are most accurate when the direction of sound wave propagation is directly in line with the direction of blood flow; that is, the insonation angle is zero. The ratio of the observed velocity to the actual velocity is the cosine of the angle of insonation.
The velocity of blood exiting either the left or right ventricle is time dependent. The integral of the velocity–time curve (VTI) can be used to estimate stroke volume if the cross-sectional area is known. Ventricular output is then the product of
The cross-sectional area is best measured at right angles to the direction of flow, which necessitates a second window for area measurement. The presence of ductal shunts reduces the usefulness of LV output as a measure of systemic flow. RV output equals venous return in the absence of atrial level shunting or anomalous pulmonary venous return, and as a consequence RV output is a better measure of systemic flow than LV output [13]. Measurement of cross-sectional area of the pulmonary outflow tract requires a significant level of skill and is usually done at the level of the insertion of the pulmonary valve leaflets.

Low-birth-weight infants and infants with significant respiratory compromise are especially prone to low systemic blood flow in the first 24 h of life. The peak velocity in the main pulmonary artery can be measured and is a useful screening tool. Peak PA velocities of greater than 0.45 m/s are unlikely to be associated with low systemic flow states, while peak velocities less than 0.35 m/s have a significant correlation with low systemic flow states [14].
Direct measures of cardiac function in the neonate, especially the preterm neonate, are complicated by the fact that the anterior wall of the LV moves much less than the lateral and posterior walls. Fractional shortening measured using LV anteroposterior diameter will be inaccurate as a consequence. For this reason a short axis view of the LV is used to derive the heart rate-corrected velocity of circumferential fiber shortening (VCFC). The relationship between VCFC and LV wall stress has been proposed as a load-independent metric of cardiac performance. The calculation of wall stress requires measuring end-systolic blood pressure, end-systolic posterior LV wall thickness, and end-diastolic LV diameter; two of these parameters must be echo derived. If VCFC is plotted against LV wall stress, there can be considerable overlap between infants with normal systemic blood flow (SBF) and those with low SBF. However, infants with myocardial dysfunction will have a greater falloff in VCFC with increases in LV wall stress than infants without myocardial dysfunction [15]. Values greater than 1.0 cirs/s at any wall stress value were almost exclusively associated with the normal SBF group [15]. Thus the absolute value of VCFC may be a useful screen for poor function, but when measured at two different wall stress values, the slope may be a better measure of function.
Echocardiography displays some limitations, most notable, the presence of an experienced echocardiographer in the neonatal ICU and/or operating room. Consequently there is a need for alternatives that are less dependent on highly trained personnel. One such device is the ultrasound cardiac output monitor (USCOM), a device that measures cardiac output from either right or left ventricle in the neonate. The device (USCOM) has been tested against echocardiographic measures in both term infants with no shunts and a small group of preterm infants. In the term infants both RV and LV outputs were measured using USCOM and echo, while only USCOM-derived output was measured in the preterm infants. In term infants, the USCOM-derived RV output was significantly greater than the echo-derived RV output, while there was better agreement between techniques for LV output [16]. The authors concluded that echo-based measures and USCOM measures were not interchangeable. As in the term infants, the USCOM-measured RV output was significantly greater than the LV output in a group of preterm infants suggesting the device systematically overestimates RV output [17]. In a subsequent publication, Meyer et al. suggested that the USCOM device undergo further controlled clinical trials before its wide application in the neonatal ICU [18].
Oscillometry:
Several oscillometry-based noninvasive blood pressure (NIBP) devices exist. Each employs the same principles but utilizes a proprietary algorithm to extract systolic, mean, and diastolic blood pressure from a signal generated by pressure fluctuations in the cuff. The amplitude of the fluctuations varies with cuff pressure. If the amplitude of the fluctuation in cuff pressure is plotted as a function of cuff pressure, the result is an oscillogram. Each oscillogram will have a unique shape and set of inflection points. The algorithms are designed to derive systolic, mean, and diastolic pressure from the data stream contained in the oscillogram.
The oscillometric pulse amplitude results from small pressure changes in the cuff due to the expansion and contraction of blood vessels within the limb encircled by the cuff as blood is ejected into the arterial tree. The diastolic pressure is related to a falloff in the rate of decrease in pulse amplitude once the peak has been achieved, and the systolic pressure is related to the steepest rate of rise of pulse amplitude after a minimum value has been crossed.
Automated noninvasive blood pressure (NIPB) greatly simplified monitoring the neonate in the operating room. There is controversy around the accuracy of NIBP especially in very-low-birth-weight infants at the low end of the blood pressure [19–22], the population most susceptible to periventricular leukomalacia, low cerebral blood flow, and inadequate peripheral perfusion. Studies have shown a systematic overestimation of mean pressure by 3–8 mmHg depending on the device used to measure NIBP [23]. The critical question is “what is the lowest tolerable pressure before target organ failure results,” a question not easily answered in the neonate. In one study of extremely low-birth-weight infants, mean arterial pressures below 23 mmHg were clearly associated with cerebral dysfunction [24]. The lower limit of blood pressures generally increases with both gestational and postnatal age; however, the lower limit of cerebral blood flow remains to be established [25].
Pulse CO-oximetry:
Advanced pulse oximetry technologies can noninvasively detect hypovolemia, hypoperfusion, and anemia, which are common situations in neonatal surgery. These conditions are difficult to monitor in neonates because insertion of arterial catheters and sampling blood remains challenging in this population.
Advances in optical technologies, especially light emitting diodes (LED), permitted the development of pulse CO-oximetry. Conventional pulse oximetry founded in the 1980s uses two LED at 660 nm and 940 nm, respectively, which were the only commercially available LED at that time. Fortunately, the absorption spectrum of oxy- and deoxyhemoglobin was well differentiated at those wavelengths which permitted the measurement of SpO2. However, carboxyhemoglobin and methemoglobin were not differentiated, and thus conventional pulse oximetry was unable to determine them or total hemoglobin concentration. In the near red and infrared region, many LED are now commercially available to permit multiwavelength pulse oximetry to measure all hemoglobin species as well as total hemoglobin concentration (SpHb), of which Masimo Corporation has been the leader. In addition, intravascular volume and perfusion status can be monitored through the plethysmograph variability index (PVI) and perfusion index (PI), which analyzes the optical signals in a different way than for hemoglobin concentration and oxygen saturation.
PI and PVI evaluate the interrelation between the cardiovascular and respiratory system to detect hemodynamic changes indicative of circulating blood volume and peripheral perfusion, analogous to respiratory variation in the pulse pressure with ventilation during invasive monitoring of the arterial waveform (Fig. 6.4a). This pulse pressure variation depends on the mode of ventilation because the effects on the venous return, right ventricle afterload, left ventricle end-diastolic pressure, and left ventricle afterload vary during spontaneous inspiration or positive pressure ventilation. This dynamic respiratory variation in arterial pulse pressure and systolic blood pressure is regarded as a sensitive indicator of circulating blood volume and as accurate as central venous pressure and pulmonary artery occlusion pressure.


Fig. 6.4
(a) Recording from a catheter in the radial artery of a mechanically ventilated adult. During positive pressure ventilation in inspiration, pulmonary flow into the left ventricle increases with a commitment increase in the left ventricular preload and decrease in the left ventricular afterload which in turn increases stroke volume and systolic blood pressure (Δ Up 2). The opposite occurs during expiration (Δ Down 3). Usually this respiratory fluctuation in the systolic blood pressure remains less than 10 mmHg, although it increases with hypovolemia, even though arterial pressure and heart rate remain normal. (b) Schematic illustration of PI and PVI. PI is the ratio of the pulsatile optical signal absorbance versus the non-pulsatile optical signal absorbance expressed as a percentage. PVI uses the optical signal variations associated with the respiratory cycle. Note similarity to (a)
Pulse index (PI) and the pleth variability index (PVI) are the optical signal components that correlate with the systolic amplitude and the systolic amplitude variation with ventilation, respectively, on the arterial waveform (Fig. 6.4b). The PI is the amplitude difference between the pulsatile and non-pulsatile optical signals and is given as a percentage of the non-pulsatile optical signal which remains constant represented by
where AC is the amplitude of the alternating pulsatile signal and DC is the amplitude of the direct, non-pulsatile signal. PI directly correlates with the strength of the pulsatile optical signal which correlates with the strength of the manual pulse (Fig. 6.4b). The PI ranges from 0.02 to 20 %, although values above 1 % generally represent normal physiologic conditions. PVI is measured from changes in the PI during a respiratory cycle. PVI is calculated by the difference between the maximum and minimum PI divided by the maximum PI during a respiratory cycle, as shown by
PVI is given in a percentage (0–100 %), in which higher PVI corresponds to higher pulse oximeter amplitude waveform difference during the respiratory cycle, which correlates with severity of hypovolemia. If hypotension is present, PVI above 12–14 % indicate hypovolemia in which intravenous fluid bolus will improve arterial pressure.


Studies in adults evaluated the clinical utility of PVI. Forget et al. [26] evaluated PVI during general anesthesia for major abdominal surgery, in which fluid management was guided by PVI in one group and not in the other group. The group that received fluid management guided by PVI had decreased blood lactate and received less intravenous crystalloid and total fluids. Canneson et al. [27] showed a good correlation between PVI and respiratory variation of pulse pressure from the invasive arterial waveform. Desebbe et al. [28] found that PVI predicted the hemodynamic changes of positive end-expiratory pressure in mechanically ventilated adults. The use of PVI in spontaneous ventilation is less clear. Keller et al. [29] showed that PVI can detect hemodynamic changes during spontaneous ventilation with passive leg raising. However, PVI did not predict response to fluid bolus for hypotension in spontaneously breathing adults.
Studies in neonates suggest that PI has clinical utility. PI immediately after birth predicted the development of chorioamnionitis, a condition associated with significant morbidity and mortality. PI had a 93.7 % positive predictive value and 100 % negative predictive value in identifying subclinical chorioamnionitis; early detection of chorioamnionitis enabled early treatment which decreased disease severity and admission to a neonatal critical care unit [30]. Similarly, PI has been evaluated to predict the severity of illness for other conditions in neonates [31]. There was an excellent correlation between the PI and the Score for Neonatal Acute Physiology with a 91.2 % positive predictive value and 96.8 % negative predictive value.
Pulse CO-oximetry detects changes in the hemoglobin concentration during surgery earlier than conventional blood draw-based laboratory measurements, decreases the number of blood samples drawn, and guides blood transfusion decisions [32]. Like conventional pulse oximetry, erroneous measurements occur during severe hyperbilirubinemia and the presence of intravascular dyes such as methylene blue. The accuracy of SpHb in healthy adult volunteers undergoing hemodilution showed a bias between SpHb and blood drawn tHb that was less than 2 g/dl for 97 % of all measurements [33]. More recently, the accuracy of SpHb in adults undergoing spine surgery showed the bias between SpHb and tHb was <2 g/dl in 77 % of patients [34]. In pediatrics, the bias and precision of SpHb compared with laboratory blood measurements were 0.9 g/dl and 1.5 g/dl, respectively [35]. An initial in vivo correction after the first measurement improved the accuracy, as SpHb trend was more accurate than absolute measurement of hemoglobin concentration. Advances in the development of sensor size will soon bring this technology for use during neonatal surgery.
The Nervous System
The neonate poses special challenges to neurological monitoring because the immaturity of the nervous system limits the extent of functional information gained by monitoring. However, the future development of the nervous system depends on protecting it during critical illness and detecting and correcting situations that may compromise its well-being. Monitoring the nervous system requires a multimodal approach including electrical activity, oxygenation, and blood flow.
EEG and Amplitude-Integrated EEG (aEEG):
The use of EEG in the neonatal intensive care unit has a long history. Due to the small size of the infant’s head, the standard montages used in adults were simplified to include recordings from 9 electrodes instead of 21. The neonatal EEG varies considerably with gestational and postnatal age. A developmental glossary of EEG in premature and full-term infant was recently published [36] and the changes were summarized [37] (Fig. 6.5). Given its complexity, interpretation of the neonatal EEG requires an experienced technician and a neurologist, which effectively limit monitoring to only a few hours a day. Amplitude-based EEG was developed in the late 1960s as an alternative tool that provided continuous EEG monitoring that could be used at the bedside by a trained nurse and a non-neurologist clinician. The cerebral function monitor, a device using amplitude-based EEG, was originally developed and studied as a tool to predict outcome following cardiac arrest in adults [38,39]. Since the publication of a reference atlas of an EEG in infants in 2003 [40], aEEG has gained popularity in both Europe and North America, as it was found to provide continuous cerebral activity information with minimal interference with care.


Fig. 6.5
Characteristic changes in EEG during the first few months of life from premature to term infant
The aEEG relies on signals from either a single pair (P3 → P4) of electrodes or two pairs (C3 → P3, C4 → P4). The central–parietal areas are preferred for monitoring the neonate as these areas are at risk for hypoperfusion from vascular watershed phenomenon. Frontal locations are not recommended for two-channel monitoring as this area is electrophysiologically underdeveloped, and seizure activity may not propagate to the frontal region [41].
The aEEG device is portable and designed for ease of use [41,42]. The signal from the electrodes is amplified, filtered (2–15 Hz band pass), rectified, and presented as a peak-to-peak voltage. By filtering out signal frequencies in excess of 15 Hz, interference from muscle activity and electrical devices is eliminated. Filtering out frequencies less than 2 Hz removes low-frequency delta waves. Many algorithms give greater weight to alpha than theta or delta waves although the predominant frequencies present in the premature neonatal EEG are in the delta and theta range. Alpha and beta emerge after 34 weeks of gestation. The aEEG is displayed at slow speeds to reveal trends. Raw EEG can be displayed on screen so that rapid changes such as seizure activity can be seen (Fig. 6.6) [41].


Fig. 6.6
Amplitude EEG patterns during various conditions: a normal aEEG trace (a) and a series of abnormal traces including discontinuous activity (b), low amplitude (c), burst suppression (d), flat trace with ECG artifact (e), and seizures (f)
The aEEG displays an upper and lower voltage band. A normal aEEG has a lower voltage greater than 5 μV and an upper value greater than 10 μV [43]. An aEEG trace with a lower band <5 μV and the upper >10 μV is moderately abnormal; the combination of lower band <5 μV and upper band <10 μV is defined as suppressed [43]. An abnormal or suppressed aEEG, when present many hours after birth asphyxia, is very predictive (>70 %) for death or neurological disability [43–45].
Changes in aEEG voltages occur with decreased cerebral perfusion or decreased cerebral metabolism. For example, aEEG amplitude decrements lasting 10–20 minutes were noted in infants given surfactant who experienced a decrease in mean blood pressure and increased pulmonary shunting [46,47]. Decreased aEEG voltages were also noted in a subgroup of infants undergoing ductus ligation under anesthesia who manifested decreases in mean blood pressure and decreases in the NIRS-measured ScO2 (see Fig. 6.7) [48].


Fig. 6.7
Amplitude EEG, near-infrared spectroscopy, and arterial pressure recordings during neonatal patent ductus ligation
Amplitude-integrated EEG patterns are related to cerebral perfusion from the first day of life, in both very premature infants and term infants with congenital heart disease [49,50]. The relationship between aEEG and blood pressure is less clear as abnormal aEEG patterns may not manifest in some infants until mean arterial pressure is less than 23 mmHg [24]. aEEG is not adversely affected by mild hypothermia [52]. Collectively, the data suggest that aEEG may be a useful monitor of the adequacy of cerebral perfusion under conditions present in the operating room. However, aEEG output must be scrutinized for artifact by examining the raw EEG as electrical interference and ECG artifact can contaminate the traces [52]. If measures are taken to minimize interference from electrical noise, the aEEG can be a valuable monitor in the operating room.
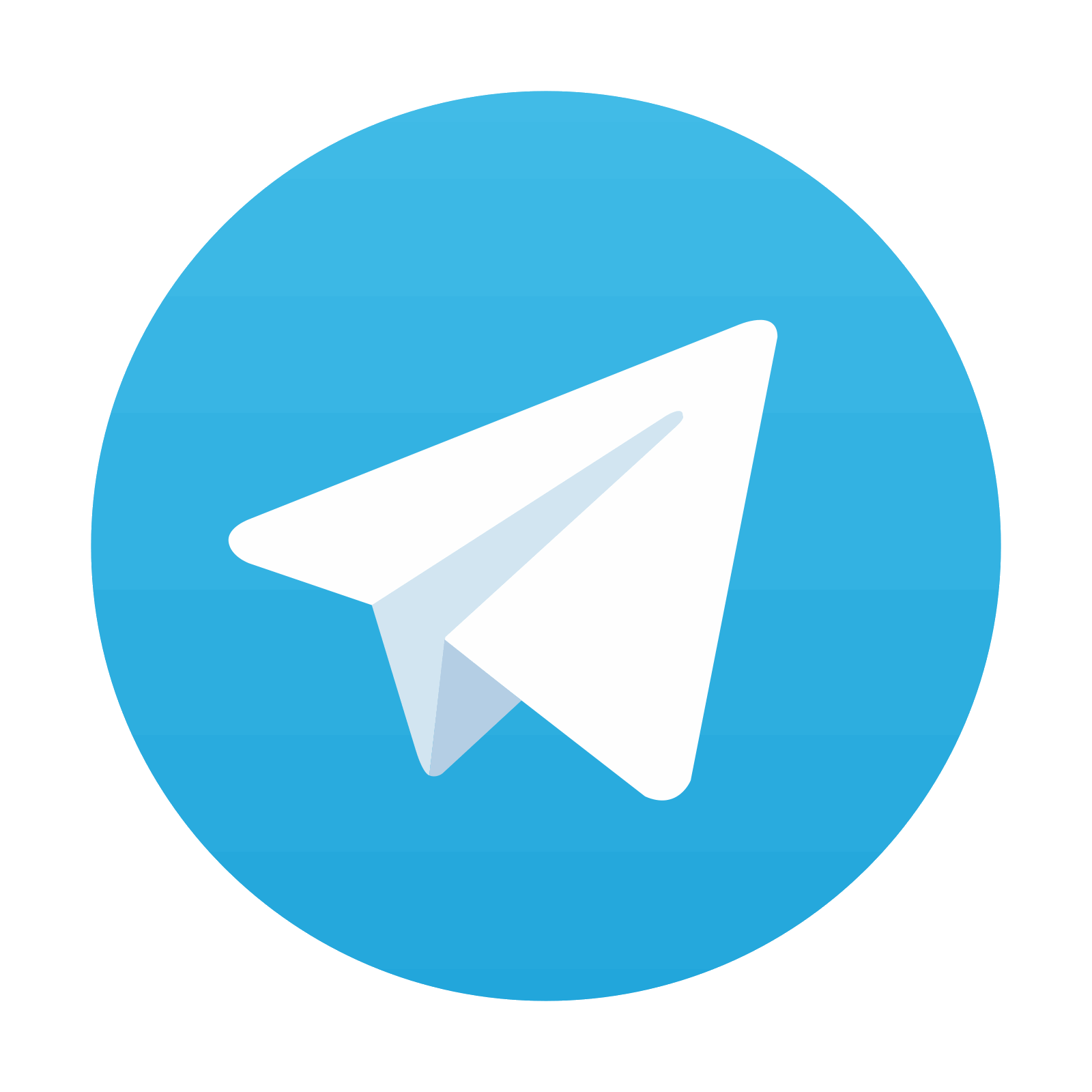
Stay updated, free articles. Join our Telegram channel
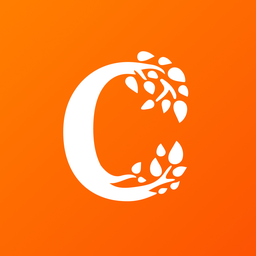
Full access? Get Clinical Tree
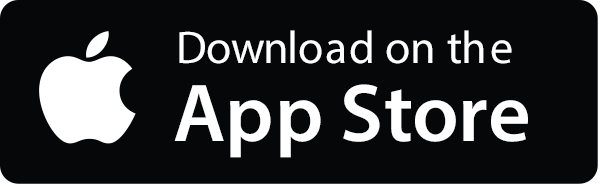
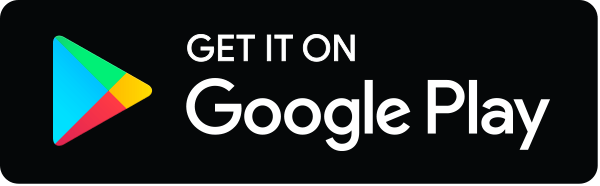