Benjamin M. Hyers, James B. Eisenkraft During anesthesia for a thoracic surgical procedure, many alterations in physiology occur, including changes in oxygenation and ventilation. Monitoring of oxygenation and ventilation are of vital importance, particularly in thoracic anesthesia because one-lung ventilation is often necessary to perform the surgery safely. Pulse oximetry, oxygen reserve index, spirometry, and capnography are the major monitoring methods the anesthesiologist should be proficient with when administering anesthesia for thoracic surgeries. oxygenation; ventilation; one-lung ventilation; pulse oximeter; capnography; compliance; dead space; spirometry During anesthesia for a thoracic surgical procedure, many alterations in physiology occur, including changes in oxygenation and ventilation. Whether the patient is receiving monitored anesthesia care (MAC), regional anesthesia, or general anesthesia, it is essential that the anesthesiologist monitor each patient diligently to optimize management and avoid complications. (“Vigilance” is the motto of the American Society of Anesthesiologists [ASA]). Monitoring of oxygenation and ventilation are of vital importance, particularly in thoracic anesthesia, where one-lung ventilation (OLV) is necessary in most cases. The anesthesiologist should be familiar with the various methods available to monitor oxygenation and ventilation, and how to correctly interpret data. The ASA has adopted two standards for basic anesthetic monitoring.1 Standard I requires that qualified anesthesia personnel be present in the procedural room over the entirety of all general anesthetics, regional anesthetics, and MAC. Standard II (Box 11.1) requires continual evaluation of a patient’s oxygenation, ventilation, circulation, and temperature during all anesthetics. This chapter will explore the various methods of monitoring oxygenation and ventilation and will emphasize their importance during thoracic anesthesia. The oxygen cascade refers to the process and pathway of oxygen being delivered from the atmosphere to a cell’s mitochondria. It consists of four steps: (1) mass transport of oxygen from the atmosphere (or breathing circuit) to the alveolar spaces; (2) diffusion of oxygen from the alveoli to the blood in the pulmonary capillaries; (3) transport of blood from the pulmonary system to the systemic circulation; (4) diffusion of oxygen from the systemic circulation to each target tissue cell. Pathology that interrupts this pathway at any of its steps can cause hypoxemia resulting in tissue and organ dysfunction.2 Monitoring a patient’s oxygenation can occur at several places in this pathway. The partial pressure of oxygen in the alveoli (PAO2) is approximately 100 mm Hg in a healthy patient breathing room air. This can be calculated by using the alveolar gas equation: where FiO2 is the fractional concentration of inspired oxygen in air (21%), Patm is atmospheric pressure (760 mm Hg at sea level), Pwater is the saturated vapor pressure of water at body temperature (47 mm Hg at 37º C), PACO2 is the partial pressure of carbon dioxide in arterial blood (40 mm Hg), and R is the respiratory quotient (usually 0.8). Hypoxemia results when there is ventilation-perfusion (V/Q) mismatch in the lungs. Regions of the lung that are ventilated but not perfused are termed dead space, whereas regions of the lung that are perfused but not ventilated are termed shunts. Dead space and shunts are the extreme opposite ends of the V/Q spectrum. Pulmonary vasculature demonstrates a phenomenon known as hypoxic pulmonary vasoconstriction (HPV), a protective mechanism that diverts blood away from parts of the lung that are not well ventilated and are hypoxic, to parts that are well ventilated in an effort to minimize V/Q mismatch.3 This is an important mechanism during thoracic surgery to reduce the transpulmonary shunt during OLV (see Ch. 15). The approximate transit time for a red blood cell to travel through the pulmonary capillary bed is 0.7 seconds. Four lung characteristics ensure an efficient transfer of oxygen from the alveoli to the pulmonary capillaries: a large oxygen partial pressure (PO2) gradient, a thin diffusion barrier with a large surface area for gas exchange, a high diffusion coefficient for oxygen, and the rapid binding of oxygen to hemoglobin. As oxygen diffuses from the alveoli into the plasma, it immediately enters an erythrocyte and is bound by hemoglobin. This ensures that the PO2 in the plasma remains low, which is essential for continued diffusion of oxygen from the alveoli into the plasma inadvertently.3 Probably the most important monitor on the anesthesia workstation is the oxygen analyzer with a low oxygen concentration alarm because it continuously monitors the concentration of oxygen (FiO2) that the patient will inspire from the breathing circuit. This is essential and should alert the anesthesiologist if a hypoxic gas mixture is inadvertently being delivered to the patient. Before oxygen analyzers were in general use, a number of complications and adverse outcomes were reported because of patients unintentionally receiving hypoxic gas mixtures.4 Such scenarios may be caused by a hypoxic gas (e.g., nitrogen, nitrous oxide) being delivered in the oxygen pipeline, failure of the “fail safe system”, failure of the nitrous oxide-oxygen proportioning system, system leaks, or low-flow anesthesia with inadequate flow of oxygen into the breathing system. The oxygen analyzer must be calibrated, and, if not performed by the workstation automatically, it is important to set the low oxygen concentration alarm limit and audible volume. The patient’s inspired gas mixture is always sampled downstream of the inspiratory unidirectional valve in the circle breathing circuit. The two most commonly used oxygen analyzers are the fuel cell and the paramagnetic oxygen analyzer.5 The fuel cell oxygen analyzer is essentially a galvanic cell that acts as an oxygen battery. Oxygen diffuses from the breathing circuit through an oxygen-permeable membrane that covers the sensor of the fuel cell. Oxygen is first reduced to negative hydroxyl ions at the cathode. The hydroxyl ions then oxidize the metal at the anode. The overall chemical reaction produces a current and creates a voltage potential that is directly proportional to the PO2 in the breathing circuit. The result is displayed on the anesthesia monitor, usually as a percentage of total inspired gas mixture. The fuel cell analyzer is calibrated by exposing it to room air (21%) at sea level. The analyzer actually measures partial pressure and not percentage. If it is calibrated to 21% at sea level and then exposed to room air (still 21% by volume) at a lower ambient pressure (i.e., at higher altitude), it will read less than 21%. The standard fuel cell oxygen analyzers are slow responding (∼30 seconds) and measure average oxygen concentrations.5 Exposure to oxygen causes the fuel cell to wear out over time and periodically it must be replaced. The paramagnetic oxygen analyzer has a very rapid response time to changing oxygen concentrations. By sampling gas at the patient’s airway (i.e., in the vicinity of the Y-piece in a circle breathing system), it provides for breath-by-breath monitoring of inspired and end-tidal oxygen concentrations. Oxygen molecules are paramagnetic and attracted to a magnetic field.6 These analyzers have two chambers, a sampling chamber and a reference chamber, separated by a sensitive pressure transducer. The reference chamber receives room air and the sampling chamber receives the gas that is being aspirated from the breathing circuit. An electromagnet rapidly switches on and off, creating a changing magnetic field that causes oxygen molecules to be attracted and agitated. This creates a pressure differential in the pressure transducer, which is directly proportional to the oxygen partial pressure difference between the sampled gas and reference gas (room air). The pressure differential is converted to a DC voltage that is directly proportional to the PO2. Paramagnetic oxygen analyzers require an in-line water trap in the gas sampling pathway because their function can be compromised by the presence of water vapor. The rapid response time of the paramagnetic oxygen analyzer allows breath-by-breath monitoring of oxygen concentration, the graphical display of which is termed the oxygram. Because oxygen and carbon dioxide (CO2) are moving in opposite directions during respiration, the oxygram is a mirror image of the capnogram. Monitoring of inspired and end-tidal oxygen concentrations is valuable in determining the efficacy of preoxygenation in a patient breathing 100% oxygen before induction of anesthesia. Ideal preoxygenation exists when the end-tidal oxygen concentration is 95% and end-tidal CO2 is 5%.6 Oxygen content in arterial blood (CaO2) has two components: oxygen that is bound to hemoglobin and oxygen that is dissolved in the blood. It is represented by Eq. 11.2. 1.34 is the oxygen combining capacity of hemoglobin (Hgb) with units of mL O2/g Hgb, Hgb is the hemoglobin concentration in units of grams of Hgb/100 mL blood, SaO2 is the percent saturation of arterial hemoglobin, 0.003 is the solubility coefficient of oxygen in units of mL O2/100 mL blood/mm Hg, and PAO2 is the partial pressure of oxygen in arterial blood in units of mm Hg. The first part of Eq. 11.2 [in the brackets] represents the oxygen that is bound to hemoglobin, and the second part of Eq. 11.2 represents oxygen dissolved in the blood. The major contributor to the total oxygen content is the oxygen bound to hemoglobin because the dissolved component has the 0.003 conversion factor. Eq. 11.2 can also be used to determine the mixed venous blood oxygen content (CvO2). By disregarding the dissolved component and assuming an SaO2 of 100%, an SvO2 (mixed venous saturation) of 75%, and a hemoglobin concentration of 15 g Hgb/100 mL blood, CaO2 is 20 mL O2/100 mL blood and CvO2 (venous oxygen content) is 15 mL O2/100 mL blood. Arterial oxygen content is directly proportional to hemoglobin concentration and hemoglobin saturation. Hemoglobin saturation and PAO2 are related by the oxygen-hemoglobin dissociation curve, which is sigmoid in shape. In adults, a PAO2 of 27 mm Hg, 40 mm Hg, and 60 mm Hg correspond to hemoglobin saturations of 50%, 75%, and 90%, respectively. Because the oxygen-hemoglobin dissociation curve is sigmoid in shape, saturations all approach 100% for PAO2 values of 90 mm Hg or higher. Certain physiologic conditions can shift the oxygen-hemoglobin dissociation curve to the right, which facilitates oxygen unloading at the tissues. These include acidosis, hypercarbia, increased 2,3-diphosphoglycerate (2,3-DPG) concentrations, and hyperthermia. The opposite of these physiologic conditions, as well as the presence of fetal hemoglobin, causes the curve to shift to the left, which increases hemoglobin’s affinity for oxygen and decreases release to the tissues. The quantity of oxygen delivered to peripheral tissues (oxygen flux) per minute can be calculated by multiplying the CaO2 by the cardiac output: O2del is the amount of oxygen delivered to tissues by the arterial blood in units of mL O2/min. The 10 is a necessary conversion factor because CaO2 has units of mL O2/100 mL blood and cardiac output has units of liters of blood per minute. Replacing CaO2 with CvO2 in Eq. 11.3 gives mL O2/min that returns to the heart. Oxygen consumption (VO2) can be calculated by subtracting oxygen returning from oxygen delivery using this form of the Fick equation: Eq. 11.4 relates oxygen consumption to cardiac output, total hemoglobin concentration, arterial hemoglobin saturation, and mixed venous saturation. Oxygenation during thoracic surgical procedures where OLV is used is determined by a variety of elements, including blood pressure, oxygen consumption, anesthetic effects on HPV, airway mechanics and reactivity, preexisting pulmonary disease, V/Q matching, and cardiac output. Significant desaturations can occur during OLV despite a high FiO2. This is caused by an obligate shunt in the nonventilated lung added to the intrapulmonary shunts present in the ventilated lung. Patients are typically placed in the lateral decubitus position for thoracotomies and thoracoscopies. In this position, rapid changes in oxygen saturation can occur secondary to compression of the mediastinal great vessels and intrapulmonary shunting.7 The pulse oximeter, a noninvasive monitor that provides continuous real-time estimates of arterial hemoglobin saturation with oxygen, can be used to warn the anesthesiologist of significant hypoxemia. It is essentially risk free to the patient and has revolutionized the practice of anesthesiology. It monitors oxygen at the level of the arterial blood, which helps to confirm that the lungs are able to transfer oxygen from the alveoli to the blood. However, the pulse oximeter does not guarantee that adequate oxygen is being delivered to, and used by, body tissues. The principles of operation of the pulse oximeter are optical plethysmography, whereby the pulsatile change in volume at the probe site is detected optically, and spectrophotometry, which determines the proportion of hemoglobin that is saturated with oxygen (oxyhemoglobin) in pulsatile arterial blood. The spectrophotometry is an application of the Beer-Lambert law, which relates solute concentrations (in this case oxygenated and deoxygenated hemoglobin) to the intensity of light transmitted through a solution (in this case, blood). The standard pulse oximeter probe contains two light emitting diodes (LEDs) and a photodetector that are typically positioned opposite each other, separated by the arterial bed being monitored (usually a fingertip or ear lobe). The LEDs emit light at 660 nm and 940 nm because deoxyhemoglobin maximally absorbs light in the 600 to 750 nm range (red band) and oxyhemoglobin maximally absorbs light in the 850 to 1000 nm range (infrared band). The LEDs are turned on and off hundreds of times per second and absorption values at each wavelength are measured by the photodetector during pulsatile flow (arterial systole) and nonpulsatile flow (diastole). The absorption values measured from pulsatile flow (the pulse-added absorbance) is considered as originating from arterial blood, whereas nonpulsatile flow is caused by venous blood and background tissue absorptions (Fig. 11.1). The photodetector measures the absorptions of light at the two wavelengths and the software divides the pulsatile flow absorption values at each wavelength by the nonpulsatile flow absorption values to produce a ratio, R. An empiric algorithm is used to relate the ratio of absorptions, R, to a saturation reading designated as SpO2. The most common locations to place pulse oximeter probes include the fingers, toes, ear lobes, and nasal ala.8
Monitoring of Oxygenation and Ventilation
Abstract
Keywords
Introduction
American Society of Anesthesiologists Standards for Basic Anesthetic Monitoring
Oxygenation
Oxygen Cascade
(Eq. 11.1)
Oxygen Analyzers
Monitoring Blood Oxygenation
(Eq. 11.2)
(Eq. 11.3)
(Eq. 11.4)
Pulse Oximetry
Principles of Operation
Stay updated, free articles. Join our Telegram channel
Full access? Get Clinical Tree
Monitoring of Oxygenation and Ventilation
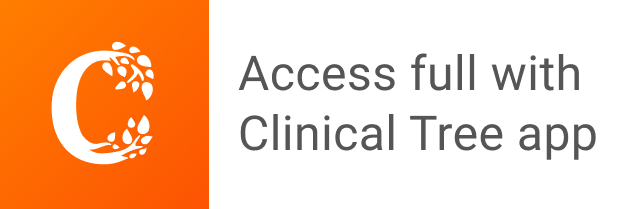