Introduction
Imaging, with its diverse array of modalities, forms an integral part of decision making in patients with neurological disorders. Diagnostic, prognostic, and pathophysiological information is provided in broadly two forms, anatomical (structural) and functional (physiologic). Anatomic or structural imaging techniques such as computed tomography (CT) and magnetic resonance imaging (MRI) provides information about normal anatomic structures of skull, meninges, brain parenchyma, vascular supply, cerebrospinal (CSF) spaces, spine, spinal cord, and spinal nerves. Information about altered anatomy is obtained in the form of intracranial hemorrhage, infarcts, fractures, tumors, aneurysms, and vascular malformations. Functional or physiologic imaging techniques like perfusion-CT, diffusion-weighted MR imaging (DWI), diffusion tensor imaging (DTI), perfusion-weighted MR imaging (PWI), and MR spectroscopy (MRS) aid in better characterizing the lesion and its effects by providing molecular, physiological, and metabolic information. This chapter will provide an overview of the imaging modalities that are used in the evaluation of central nervous system (CNS) lesions. Then, we discuss representative neurological disorders and the role of recent technological advancements in their evaluation.
Imaging modalities
Structural Imaging Modalities
Plain Radiographs
Though plain radiographs were used for a lot of neurodiagnostic work, especially trauma patients, the advent of multiplanar imaging techniques, mainly CT and MRI, has relegated that role to the confines of history. Skull or spine radiographs can detect fractures; however, sensitivity is less than that of CT and intracranial and intraspinal injury cannot be as accurately evaluated by plain radiographs. Besides trauma, one practical use for skull radiographs which is still used in present settings is to exclude metallic foreign body in orbits prior to MRI.
Computed Tomography
It is not wrong to say that CT is the workhorse of a modern neurodiagnostic imaging facility, especially in emergent settings. Its widespread availability and short scan time make it the preferred imaging modality for initial evaluation of many intracranial lesions. The short scan time makes it especially useful in the settings of stroke and trauma in agitated, unstable patients who can benefit tremendously from the early detection of and neurosurgical intervention in incidences such as intracranial hemorrhage, hydrocephalus, and impending herniation. The early detection prompts early surgical intervention, which has been documented as improving patient outcomes. This has resulted in the increased utilization of CT scans in emergency departments over the last decade. Another advantage is volumetric data acquisition, which helps in better evaluation of intracranial and spinal structures in three dimensions. The principal disadvantage of CT is the ionizing radiation exposure, which has prompted, among other things, a campaign among clinicians and radiologists, the “Image Gently” campaign, to popularize a means of reducing the radiation dose in pediatric settings. One of the other disadvantages of CT is its inability to assess lesions in areas such as the posterior fossa and the floor of the middle cranial fossa due to “beam hardening” artifacts resulting from the attenuation of the X-ray beam behind relatively dense structures such as the clivus and temporal bone. Contrast agents commonly used in CT are iodine-based, the majority now being nonionic, resulting in lesions being more conspicuous due to breakdown of the blood–brain barrier.
Magnetic Resonance Imaging
MRI is increasingly being used for the delineation of details of the anatomy and also of complex lesions of the central nervous system. It uses the signals emitted by the relaxation of ubiquitous hydrogen nuclei present in water after they have been excited by radiofrequency pulses in a strong magnetic field. By varying the different pulse sequences in obtaining images, the soft tissue contrast of visualized anatomical structures can be varied. On T1-weighted images, fat appears bright and water and cerebrospinal fluid (CSF) appear black. On T2-weighted images, fat has intermediate signal appearing gray, while water and CSF appear bright. Cortical bone, composed of relatively fixed protons, does not produce a signal. Flowing blood produces no signal resulting in a so-called “signal void.” In general, pathologic processes usually contain excess amounts of free water and therefore are dark on T1-weighted images and bright on T2-weighted images. Hence, the rule of thumb is that anatomy is better seen on T1-weighted and pathology better on T2-weighted sequences. Contrast agents in the majority of cases are paramagnetic (eg, gadolinium-DTPA) and result in vessels and pathologic lesions being more conspicuous due to an increased T1 signal. This is the reason that post-contrast sequences are T1-weighted.
Different pulse sequences can be used for different types of CNS lesions. One of the commonest sequences to be used in all brain MRIs is the DWI sequence, which is particularly helpful in detecting early ischemia. This we shall discuss further in the functional neuroimaging section. Fluid-attenuated inversion recovery (FLAIR) sequence increases the conspicuity of focal hyperintense T2 signal in pathologic lesions by eliminating (or “nulling”) the hyperintense CSF signal. Thus, focal bright gray matter abnormalities in contusions or white matter abnormalities in diffuse axonal injuries (DAI) or multiple sclerosis are more easily appreciated against the adjacent “nulled” dark CSF spaces. FLAIR also has increased sensitivity for the presence of acute or subacute subarachnoid hemorrhage (SAH), which appears as a hyperintense signal within the sulci and cisterns. The gradient-echo (GRE) T2*-weighted MR sequence shows increased sensitivity for detection of intracranial blood. However, susceptibility-weighted imaging (SWI) has now proven to be more sensitive and better in detecting hemorrhage and calcification.
Magnetic resonance safety protocols and extensive pre-procedural screening are needed for the safety of the patients with the increasing use of biomedical implants and devices. Cardiac pacemakers, intraocular metallic fragments, mechanical device implants such as cochlear implants, drug infusion pump, neurostimulators for deep brain stimulation, and ferromagnetic aneurysm clips are considered a contraindication to MR imaging. Nonferromagnetic or weakly ferromagnetic aneurysm clips such as those of titanium alloy or pure titanium have been shown to be MR compatible and safe. Similar ferromagnetic objects may pose a risk of adverse effects including magnetically induced movements resulting in dislodgement and injury to organs, current, and heating. Of secondary concern is the fact that the ferromagnetic materials can produce image artifacts, thereby degrading the image quality.
Conventional Cerebral Angiography
In conventional angiography, a flexible catheter is introduced through the right femoral artery to visualize the intracranial arteries. Other less commonly used sites for access include left femoral artery, axillary, and brachial artery. Mild intravenous sedation improves patient comfort and cooperation, and reduces anxiety. The clinical question and disease process generally determines which vessels need to be examined. Routinely a four-vessel angiogram is performed, in which bilateral internal carotid and vertebral arteries are investigated. In a six-vessel angiogram, both external carotid arteries are also studied. The cerebral vasculature is visualized by injecting an iodinated contrast agent into these arteries and subsequently performing digital subtraction angiography (DSA), in which bony details are subtracted from a “mask” image, thus leaving us with images of different vessels. Serial fluoroscopic spot images are obtained throughout the procedure and standard angiographic views of the vessel of interest, including antero-posterior, lateral, and, if needed, oblique, are obtained. This principle can be extended for application in endovascular surgery, where cerebral angiographic techniques can not only be of diagnostic value, but also provide a route for endovascular therapy in entities such as cerebral aneurysms, arteriovenous malformation, and tumor embolization.
Cerebral angiography is considered to be the gold standard for the evaluation of cerebrovascular diseases. But it is an invasive procedure with the associated procedural risk of neurological complications—0.3–1.3%, of which 0.07–0.5% are permanent complications. , The majority of these complications are minor and transient including groin hematomas, femoral artery injury, and minor allergic reactions. However, more severe complications occasionally occur, such as cerebral infarction, seizure, and death. Spinal angiography poses the same risks as cerebral angiography, with the added danger of cord infarction secondary to spinal artery embolus. For this reason, spinal angiography should only be performed when a vascular malformation is demonstrated by another imaging modality or when the patient has SAH with normal cerebral angiography and a spinal source is strongly suspected. With improvements in the detection rates of cerebrovascular disease with newer, relatively noninvasive modalities such as CT angiography (CTA) or MR angiography (MRA), conventional catheter-based DSA has been replaced by CTA or MRA in some centers as the screening and diagnostic tool for intracranial vascular disease.
Magnetic Resonance Angiography and Computed Tomography Angiography
MRA is noninvasive and offers the opportunity to accurately depict the intracranial vasculature from different angles using a variety of methods for obtaining angiographic data. Three different MRA techniques are routinely available: time- of-flight MRA (TOF MRA), phase-contrast MRA (PC MRA), and contrast-enhanced MRA (CE MRA). The simplest and most widely used approach, TOF MRA, relies on in-flow enhancement. Essentially, static tissue within a two- or three-dimensional slice gives a low signal due to the saturating effect of the long train of closely spaced excitation pulses used in forming the image. On flowing into the imaging volume, unsaturated blood appears hyperintense relative to the static surround. , Three-dimensional TOF MRA is recommended for arterial evaluation, and a two-dimensional TOF MRA technique for venous evaluation. The disadvantages of MRA include limited visualization of very small distal cortical or deep branches, and a dependence on flow or the patient’s cooperation. To better visualize the veins and small arterial branches, intravenous contrast enhanced MRA can be used, but with the disadvantages of increased cost, and superimposition of veins and of enhanced soft tissues.
Advances in multidetector row CT (MDCT) have facilitated the quick and accurate examination of the cerebral vasculature. CTA is widely available, with fast, thin-section, volumetric spiral CT images acquired during the injection of a time-optimized bolus of contrast material for vessel opacification. With modern multisection CT scanners, the entire region from the aortic arch up to the circle of Willis can be covered in a single data acquisition, with excellent, three-dimensional spatial resolution. Multiplanar reformatted images, maximum intensity projection images (MIP), and three-dimensional reconstructions of axial CTA source images combine to provide images comparable, or even superior, to those obtained with conventional angiography ( Fig. 5.1 ).
Myelography and Computed Tomography Myelography
Myelography involves the injection of iodinated, water-soluble contrast material into the spinal subarachnoid space via a lumbar or, very rarely, a lateral C1–C2 puncture. Plain radiographs are obtained in multiple projections while the contrast is moved cranially or caudally to evaluate multiple levels. Better contrast agents and smaller-gauge spinal needles now permit myelography to be performed on an outpatient basis. After the study, the contrast agent is left in the subarachnoid space, from which it is absorbed and excreted in the urine. Myelography is typically followed by immediate or delayed CT examination (CT myelography). Nonionic contrast agents have dramatically reduced the side effects and complications associated with myelography. The most common post-procedural complication is mild-to-severe headache with nausea and/or vomiting. Because contrast material lowers the seizure threshold, patients with a history of seizures should be studied cautiously and patients on medications known to lower the seizure threshold (eg, tricyclic antidepressants) are commonly instructed to stop taking the drug 3 days before the myelography, to allow adequate drug clearance.
A CT myelogram has all the features of routine CT, including better spatial resolution with the added benefit of radio-opaque contrast material outlining the spinal cord and nerve roots. Pathologic lesions appear as abnormal filling defects within the contrast column, and any cord enlargement or deviation from anatomic position is easily appreciated ( Fig. 5.2 ). Now, MRI has become the imaging spine modality of choice, superseding both myelography and CT myelography, for the evaluation of spine pathology. The capability for multiplanar imaging, the use of nonionizing radiation, and the ability to obtain a myelographic-like image without an intrathecal contrast injection all provide distinct advantages. But still, myelography is preferred by some surgeons and is better in many circumstances, including postsurgical cases where MR quality is severely degraded due to artifacts from hardware.
Functional Imaging Modalities
Functional or physiologic imaging provides complementary information to structural imaging and thus facilitates a better characterization of CNS pathology. Imaging of cerebral function can potentially define the early pathophysiological processes responsible for neuronal injury, assess the efficacy of therapeutic interventions, and direct the design and implementation of future therapeutic interventions aimed at reversing or preventing neuronal injury.
Perfusion Computed Tomography
Based on the multicompartmental tracer kinetic model, dynamic perfusion CT imaging is performed by monitoring the first pass of an iodinated contrast agent bolus through the cerebral circulation ( Fig. 5.3 ). As the change in CT enhancement (in Hounsfield units, HU) is proportional to the concentration of contrast, perfusion parameters are calculated by deconvolution from the changes in the density–time curve for each pixel using mathematical algorithms based around the central volume principle: ,
- 1.
Mean transit time (MTT) indicates the time difference between the arterial inflow and venous outflow.
- 2.
Time to bolus peak (TTP) indicates the time from the beginning of contrast material injection to the maximum (peak) concentration of contrast material within a region of interest.
- 3.
Cerebral blood volume (CBV) indicates the volume of blood per unit of brain mass (normal range in gray matter, 4–6 mL/100 g).
- 4.
Cerebral blood flow (CBF) indicates the volume of blood flow per unit of brain mass per minute (normal range in gray matter, 50–60 mL/100 g/min). The relationship between CBF and CBV is expressed by the equation CBF = CBV/MTT.
The main advantage of perfusion-CT is its wide availability and quantitative accuracy. Its main limitation is its inability to image the whole brain, as it is limited to a 2–3 cm section of brain tissue per bolus. Now with the availability of 320-slice volumetric multimodal CT, it is possible to visualize dynamic changes in the blood flow of the entire brain.
Diffusion-weighted Magnetic Resonance Imaging and Diffusion Tensor Imaging
DWI is based on the measurement of the random (Brownian) motion of water molecules and detects the degree of mobility (or diffusibility) of water molecules within tissues. By introducing spatial magnetic field gradients, it is possible to obtain MR sequences that are sensitive to the diffusivity of water along a chosen direction, obtaining so-called DWI. From the ratio of DWI images acquired at different levels of diffusion sensitization (known as b-value), it is possible to compute a quantitative measure of mean diffusivity, known as the apparent diffusion coefficient (ADC). The ADC measures water diffusion and, therefore, often mirrors changes in the DWI signal. In areas of increased diffusion like vasogenic edema, DWI signal intensity is low, and there is an increase in ADC. In regions of restricted diffusion like cytotoxic edema, DWI signal intensity is increased, and the ADC signal decreases.
This technique is widely used in acute ischemic stroke in which reduced diffusion resulting in increased DWI signal and reduced ADC is seen before the onset of visible abnormalities on conventional MR imaging. , In the affected region, there is a temporal evolution from restricted diffusion (ie, cytotoxic edema in the acute stroke setting) to unrestricted diffusion (ie, vasogenic edema and encephalomalacia in the chronic setting). DWI is also used to study other CNS processes such as cerebral and spinal abscesses, epidermoid cysts, traumatic brain injury (TBI), , and studying brain maturation and development, especially the myelination process.
Water diffusion properties also play a role in DTI—a related imaging technique. Whereas gray matter is microstructurally compact with no directional preference, white matter bundles are arranged in a highly directional manner. For this reason, while water usually diffuses in all directions (meaning diffusion is isotropic) in the brain, water diffuses preferentially along white matter tracts rather than across them (meaning diffusion in white matter is anisotropic). A mathematical algorithm known as diffusion tensor is commonly used to model anisotropic diffusion in white matter. Fractional anisotropy (FA) maps can be created—FA index varies between 0 (representing a symmetrical anisotropic medium where there is no directionality of the diffusion, for instance in water) and 1 (representing maximum anisotropy). Also, directionally encoded color maps and three-dimensional tractography can be performed to visually display white matter tracts ( Fig. 5.4 ).
Perfusion-weighted Magnetic Resonance Imaging
While DWI is most useful for detecting irreversibly infarcted tissue, PWI may be used to identify areas of reversible ischemia as well. PWI techniques rely either on an exogenous method of achieving perfusion contrast (ie, the administration of an MR contrast agent typically gadopentate dimeglumine [Gd-DTPA, Magnevist®]) or on an endogenous method, which uses an endogenous diffusible tracer to measure CBF by applying magnetic resonance pulses to tag in-flowing water protons. , PWI is most commonly applied as bolus tracking following the intravenous administration of a bolus of gadolinium contrast. The passage of the contrast agent through the brain capillaries causes a transient loss of signal because of the susceptibility (T2*) effects of the contrast agent. A hemodynamic time-signal intensity curve is produced with subsequent calculation of MTT, TTP, CBF, and CBV perfusion maps using the same principles as those underlying perfusion CT imaging ( Fig. 5.5 ). , PWI can also be performed using T1-weighted imaging, also following an injection of gadolinium contrast. This technique requires a longer acquisition, but can measure the permeability of the blood–brain barrier.
Brain perfusion can also be assessed using another MRI technique called arterial spin labeling (ASL) ( Fig. 5.6 ). This method does not use an exogenous contrast agent, but rather uses an endogenous diffusible tracer to measure perfusion parameters by applying MR pulses to magnetically labeled in-flowing water protons. With increasing computer processing speeds and the speed of processing involved, this method is being increasingly used in clinical settings moving slowly away from the domain of being a purely research tool. This method is especially useful in patients who cannot be administered with contrast due to renal failure.
Magnetic Resonance Spectroscopy
MRS allows noninvasive, in vivo assessment of brain metabolism. The physical basis of MRS is the chemical shift effect. It actually means that nuclei located in different molecular environments sense slightly different magnitudes of magnetic field, causing them to process at different rates. MRS provides plots or spectra of signal intensity, which is proportional to concentration, versus precession rate shift with respect to a reference, expressed in parts per million (ppm). Various biologically relevant metabolites can be identified based on subtly different resonant frequencies, which are a reflection of their specific chemical environment. These metabolites reflect aspects of neuronal integrity, energy metabolism, and cell membrane proliferation or degradation. In clinical practice, five major metabolites containing hydrogen nuclei (1H-MRS) are typically evaluated ( Fig. 5.7 ): ,
- 1.
Creatine/phosphocreatine (Cr/PCr), 3.04 ppm: Creatine and phosphocreatine are involved in cellular energy metabolism and ATP production. As creatine and phosphocreatine levels tend to be relatively constant in the normal brain, they are used as a reference metabolite with the concentrations of other metabolites expressed as the ratio of the peak areas compared with the creatine peak.
- 2.
N-acetyl aspartate (NAA), 2.02 ppm: NAA is a cellular amino acid and is a neuronal marker and a measure of neuronal density and integrity. Reduced levels have been reported in a wide spectrum of conditions involving neuronal death or dysfunction, decreased neural metabolism, axonal/dendritic loss, reduced myelination. As most brain tumors are of non-neuronal origin, NAA is absent or greatly reduced, as it is with other insults to the brain such as infarction or demyelination that produce neuronal dysfunction or loss. A decrease in NAA has also been observed after head injury.
- 3.
Choline (Cho), 3.24 ppm: Cho is a composite signal of choline compounds (glycerophosphocholine [GPC], phosphocholine [PC] and a small amount of free choline) and thus reflects total brain choline stores. Cho is a constituent of the phospholipid metabolism of cell membranes and reflects membrane turnover. A Cho increase is characteristic of brain tumors due to accelerated membrane turnover in rapidly dividing cancer cells.
- 4.
Lactate (Lac), 1.33 ppm: Under normal conditions, lactate is barely detectable by MRS in the normal healthy brain. Increased lactate production occurs in disorders of energy metabolism and suggests altered energy metabolism and is consistent with cerebral ischemia.
- 5.
Glutamate and glutamine (Glx), composite peak between 2.1 and 2.5 ppm: Glutamate is an excitatory neurotransmitter that plays a role in mitochondrial metabolism. Gamma-aminobutyric acid is an important product of glutamate. Glutamine plays a role in detoxification and regulation of neurotransmitter activities. Elevated glutamate levels lead to excitotoxic cell damage. Increased glutamine synthesis occurs as a result of increased blood ammonia levels.
Intraoperative Magnetic Resonance Imaging
The use of intraoperative MRI monitoring, a blend of MR imaging performed in an operative suite, is becoming increasingly common for precise navigation and resection of various intracranial and spinal lesions. Hence, understanding the working of intraoperative MRI imaging suite is important for contemporary neuroanesthesiologists.
In 65–92% of cases in which neurosurgeons thought that they had achieved gross total resection, intraoperative MRI showed tumor that could still be resected. Prior to the development of intraoperative MRI, various stereotactic navigational systems had been used for better localization and precision of resection. The first operating theater with an MRI was built at Brigham and Women’s Hospital in 1994, and since then this technology has proliferated and has been adopted by many centers worldwide. The “hostile” MRI environment poses many limitations on patient management. This includes MRI-compatible or MRI-safe equipment, electromagnetic interference on monitoring equipment, and reduced access to the patient, all issues of importance for the anesthesiologist.
Three types of intraoperative MRI systems are in use. The original one consisted of an open system with a stationary magnet and stationary patient. However, it had limitations in the ease of access for surgeons and anesthesiologists, and limits regarding the specific instruments that could be used for surgery and monitoring. More commonly now, the other two systems consist of stationary magnet/movable patient and movable magnet/stationary patient. The most common type involves a movable magnet and a stationary patient ( Fig. 5.8 ). In this MRI setting, incompatible surgical instruments can be used. However, a drawback is that image acquisition can be done only after the patient has been placed in the magnet, which means increased time and also the need to maintain a sterile field during imaging. The cost of developing such rooms also increases as there is need for specialized MRI shielding. The use of a stationary magnet and movable patient system has similar advantages and disadvantages to the movable magnet/stationary patient system. However, there is the additional advantage of including other imaging modalities such as positron imaging tomography and biplanar fluoroscopy.
During preoperative assessment, history of acquired or implanted metallic devices, such as cerebrovascular clips, cochlear implants, cardiac pacemakers, intravascular wires, stents, bullets, extensive tattoos, and permanent eye make-up needs to be elicited. Displacement or dislodgement may occur as these devices are ferromagnetic. Braces or dentures also generate significant artifacts and degrade obtained images. Heating of metallic implants can lead to severe burns. Familiarity with the concept of safety zones, which are common to all MRI environments and were devised by the American College of Radiology (ACR), , is important in order to design processes at each stage of patient interaction in intraoperative MRI that facilitate optimal patient care. The ACR has divided the zones into four, with zone I being a general access area and zone IV within a high-strength magnetic field, with increasingly restricted access.
The use of intraoperative MRI also puts significant limitations on the equipment used, the patient, and the overall environment. An important concept in this regard is the 5 Gauss (5-G) line. The area within this line has a static magnetic field greater than 5G. Static magnetic field exposure of 5G or less is considered to be a minimal risk to bystanders. In the intraoperative MRI suite, a clearly designated 5-G line and the use of MR safe devices is encouraged. In this regard, MRI safe means the item poses no risk in the MRI environment. MRI unsafe means that the item poses a hazard in all MRI environments and is contraindicated within zone IV. MRI conditional means that a given item has been shown to pose no known hazards in a specified MRI with particular conditions of use. An example is an MRI conditional anesthesia machine that is conditional to 100 Gauss in a 1.5 Tesla magnet, but unsafe in strengths higher than this, such as a 3 Tesla field strength.
An MRI-compatible anesthesia machine, infusion pumps, pulse oximeter, end-tidal gas analyzer, electrocardiogram, blood pressure, and temperature monitoring devices are needed so that the patient does not suffer any injury such as a burn from MRI-unsafe leads. Inadvertent ferromagnetic substances become projectile in a high-strength field and monitoring equipment does not interfere with image quality. MR safe/conditional items such as defibrillators, fluid-warming devices, forced air-warming devices, Doppler ultrasound machines, peripheral nerve stimulators, and core temperature probes can be used in the nonimaging portion of the procedure when the patient is in zone III. Small children, especially neonates who have a limited ability to self-regulate temperature, need hot-air or fluid warmers, which are MR unsafe and need to be discontinued during imaging portions when the patient is placed in zone IV. The patient must be covered in sterile drapes during this portion to maintain temperature.
A recent study found the average length of surgery was increased by 1 hour 47 minutes when comparing intraoperative MRI with conventional nonintraoperative MRI neurosurgical resection of intracranial lesions. In 42% of cases, imaging led to further tissue resection. There was an increase in early reoperation (within 2 weeks of surgery) in 7.7% in the nonintraoperative MRI group compared with 0% in the intraoperative MRI group. The authors felt that despite the cost and increased surgery time, the lower early reoperation rate and patient benefits justified intraoperative MRI usage and potentially lowered the costs in the long term.
Imaging modalities
Structural Imaging Modalities
Plain Radiographs
Though plain radiographs were used for a lot of neurodiagnostic work, especially trauma patients, the advent of multiplanar imaging techniques, mainly CT and MRI, has relegated that role to the confines of history. Skull or spine radiographs can detect fractures; however, sensitivity is less than that of CT and intracranial and intraspinal injury cannot be as accurately evaluated by plain radiographs. Besides trauma, one practical use for skull radiographs which is still used in present settings is to exclude metallic foreign body in orbits prior to MRI.
Computed Tomography
It is not wrong to say that CT is the workhorse of a modern neurodiagnostic imaging facility, especially in emergent settings. Its widespread availability and short scan time make it the preferred imaging modality for initial evaluation of many intracranial lesions. The short scan time makes it especially useful in the settings of stroke and trauma in agitated, unstable patients who can benefit tremendously from the early detection of and neurosurgical intervention in incidences such as intracranial hemorrhage, hydrocephalus, and impending herniation. The early detection prompts early surgical intervention, which has been documented as improving patient outcomes. This has resulted in the increased utilization of CT scans in emergency departments over the last decade. Another advantage is volumetric data acquisition, which helps in better evaluation of intracranial and spinal structures in three dimensions. The principal disadvantage of CT is the ionizing radiation exposure, which has prompted, among other things, a campaign among clinicians and radiologists, the “Image Gently” campaign, to popularize a means of reducing the radiation dose in pediatric settings. One of the other disadvantages of CT is its inability to assess lesions in areas such as the posterior fossa and the floor of the middle cranial fossa due to “beam hardening” artifacts resulting from the attenuation of the X-ray beam behind relatively dense structures such as the clivus and temporal bone. Contrast agents commonly used in CT are iodine-based, the majority now being nonionic, resulting in lesions being more conspicuous due to breakdown of the blood–brain barrier.
Magnetic Resonance Imaging
MRI is increasingly being used for the delineation of details of the anatomy and also of complex lesions of the central nervous system. It uses the signals emitted by the relaxation of ubiquitous hydrogen nuclei present in water after they have been excited by radiofrequency pulses in a strong magnetic field. By varying the different pulse sequences in obtaining images, the soft tissue contrast of visualized anatomical structures can be varied. On T1-weighted images, fat appears bright and water and cerebrospinal fluid (CSF) appear black. On T2-weighted images, fat has intermediate signal appearing gray, while water and CSF appear bright. Cortical bone, composed of relatively fixed protons, does not produce a signal. Flowing blood produces no signal resulting in a so-called “signal void.” In general, pathologic processes usually contain excess amounts of free water and therefore are dark on T1-weighted images and bright on T2-weighted images. Hence, the rule of thumb is that anatomy is better seen on T1-weighted and pathology better on T2-weighted sequences. Contrast agents in the majority of cases are paramagnetic (eg, gadolinium-DTPA) and result in vessels and pathologic lesions being more conspicuous due to an increased T1 signal. This is the reason that post-contrast sequences are T1-weighted.
Different pulse sequences can be used for different types of CNS lesions. One of the commonest sequences to be used in all brain MRIs is the DWI sequence, which is particularly helpful in detecting early ischemia. This we shall discuss further in the functional neuroimaging section. Fluid-attenuated inversion recovery (FLAIR) sequence increases the conspicuity of focal hyperintense T2 signal in pathologic lesions by eliminating (or “nulling”) the hyperintense CSF signal. Thus, focal bright gray matter abnormalities in contusions or white matter abnormalities in diffuse axonal injuries (DAI) or multiple sclerosis are more easily appreciated against the adjacent “nulled” dark CSF spaces. FLAIR also has increased sensitivity for the presence of acute or subacute subarachnoid hemorrhage (SAH), which appears as a hyperintense signal within the sulci and cisterns. The gradient-echo (GRE) T2*-weighted MR sequence shows increased sensitivity for detection of intracranial blood. However, susceptibility-weighted imaging (SWI) has now proven to be more sensitive and better in detecting hemorrhage and calcification.
Magnetic resonance safety protocols and extensive pre-procedural screening are needed for the safety of the patients with the increasing use of biomedical implants and devices. Cardiac pacemakers, intraocular metallic fragments, mechanical device implants such as cochlear implants, drug infusion pump, neurostimulators for deep brain stimulation, and ferromagnetic aneurysm clips are considered a contraindication to MR imaging. Nonferromagnetic or weakly ferromagnetic aneurysm clips such as those of titanium alloy or pure titanium have been shown to be MR compatible and safe. Similar ferromagnetic objects may pose a risk of adverse effects including magnetically induced movements resulting in dislodgement and injury to organs, current, and heating. Of secondary concern is the fact that the ferromagnetic materials can produce image artifacts, thereby degrading the image quality.
Conventional Cerebral Angiography
In conventional angiography, a flexible catheter is introduced through the right femoral artery to visualize the intracranial arteries. Other less commonly used sites for access include left femoral artery, axillary, and brachial artery. Mild intravenous sedation improves patient comfort and cooperation, and reduces anxiety. The clinical question and disease process generally determines which vessels need to be examined. Routinely a four-vessel angiogram is performed, in which bilateral internal carotid and vertebral arteries are investigated. In a six-vessel angiogram, both external carotid arteries are also studied. The cerebral vasculature is visualized by injecting an iodinated contrast agent into these arteries and subsequently performing digital subtraction angiography (DSA), in which bony details are subtracted from a “mask” image, thus leaving us with images of different vessels. Serial fluoroscopic spot images are obtained throughout the procedure and standard angiographic views of the vessel of interest, including antero-posterior, lateral, and, if needed, oblique, are obtained. This principle can be extended for application in endovascular surgery, where cerebral angiographic techniques can not only be of diagnostic value, but also provide a route for endovascular therapy in entities such as cerebral aneurysms, arteriovenous malformation, and tumor embolization.
Cerebral angiography is considered to be the gold standard for the evaluation of cerebrovascular diseases. But it is an invasive procedure with the associated procedural risk of neurological complications—0.3–1.3%, of which 0.07–0.5% are permanent complications. , The majority of these complications are minor and transient including groin hematomas, femoral artery injury, and minor allergic reactions. However, more severe complications occasionally occur, such as cerebral infarction, seizure, and death. Spinal angiography poses the same risks as cerebral angiography, with the added danger of cord infarction secondary to spinal artery embolus. For this reason, spinal angiography should only be performed when a vascular malformation is demonstrated by another imaging modality or when the patient has SAH with normal cerebral angiography and a spinal source is strongly suspected. With improvements in the detection rates of cerebrovascular disease with newer, relatively noninvasive modalities such as CT angiography (CTA) or MR angiography (MRA), conventional catheter-based DSA has been replaced by CTA or MRA in some centers as the screening and diagnostic tool for intracranial vascular disease.
Magnetic Resonance Angiography and Computed Tomography Angiography
MRA is noninvasive and offers the opportunity to accurately depict the intracranial vasculature from different angles using a variety of methods for obtaining angiographic data. Three different MRA techniques are routinely available: time- of-flight MRA (TOF MRA), phase-contrast MRA (PC MRA), and contrast-enhanced MRA (CE MRA). The simplest and most widely used approach, TOF MRA, relies on in-flow enhancement. Essentially, static tissue within a two- or three-dimensional slice gives a low signal due to the saturating effect of the long train of closely spaced excitation pulses used in forming the image. On flowing into the imaging volume, unsaturated blood appears hyperintense relative to the static surround. , Three-dimensional TOF MRA is recommended for arterial evaluation, and a two-dimensional TOF MRA technique for venous evaluation. The disadvantages of MRA include limited visualization of very small distal cortical or deep branches, and a dependence on flow or the patient’s cooperation. To better visualize the veins and small arterial branches, intravenous contrast enhanced MRA can be used, but with the disadvantages of increased cost, and superimposition of veins and of enhanced soft tissues.
Advances in multidetector row CT (MDCT) have facilitated the quick and accurate examination of the cerebral vasculature. CTA is widely available, with fast, thin-section, volumetric spiral CT images acquired during the injection of a time-optimized bolus of contrast material for vessel opacification. With modern multisection CT scanners, the entire region from the aortic arch up to the circle of Willis can be covered in a single data acquisition, with excellent, three-dimensional spatial resolution. Multiplanar reformatted images, maximum intensity projection images (MIP), and three-dimensional reconstructions of axial CTA source images combine to provide images comparable, or even superior, to those obtained with conventional angiography ( Fig. 5.1 ).
Myelography and Computed Tomography Myelography
Myelography involves the injection of iodinated, water-soluble contrast material into the spinal subarachnoid space via a lumbar or, very rarely, a lateral C1–C2 puncture. Plain radiographs are obtained in multiple projections while the contrast is moved cranially or caudally to evaluate multiple levels. Better contrast agents and smaller-gauge spinal needles now permit myelography to be performed on an outpatient basis. After the study, the contrast agent is left in the subarachnoid space, from which it is absorbed and excreted in the urine. Myelography is typically followed by immediate or delayed CT examination (CT myelography). Nonionic contrast agents have dramatically reduced the side effects and complications associated with myelography. The most common post-procedural complication is mild-to-severe headache with nausea and/or vomiting. Because contrast material lowers the seizure threshold, patients with a history of seizures should be studied cautiously and patients on medications known to lower the seizure threshold (eg, tricyclic antidepressants) are commonly instructed to stop taking the drug 3 days before the myelography, to allow adequate drug clearance.
A CT myelogram has all the features of routine CT, including better spatial resolution with the added benefit of radio-opaque contrast material outlining the spinal cord and nerve roots. Pathologic lesions appear as abnormal filling defects within the contrast column, and any cord enlargement or deviation from anatomic position is easily appreciated ( Fig. 5.2 ). Now, MRI has become the imaging spine modality of choice, superseding both myelography and CT myelography, for the evaluation of spine pathology. The capability for multiplanar imaging, the use of nonionizing radiation, and the ability to obtain a myelographic-like image without an intrathecal contrast injection all provide distinct advantages. But still, myelography is preferred by some surgeons and is better in many circumstances, including postsurgical cases where MR quality is severely degraded due to artifacts from hardware.
Functional Imaging Modalities
Functional or physiologic imaging provides complementary information to structural imaging and thus facilitates a better characterization of CNS pathology. Imaging of cerebral function can potentially define the early pathophysiological processes responsible for neuronal injury, assess the efficacy of therapeutic interventions, and direct the design and implementation of future therapeutic interventions aimed at reversing or preventing neuronal injury.
Perfusion Computed Tomography
Based on the multicompartmental tracer kinetic model, dynamic perfusion CT imaging is performed by monitoring the first pass of an iodinated contrast agent bolus through the cerebral circulation ( Fig. 5.3 ). As the change in CT enhancement (in Hounsfield units, HU) is proportional to the concentration of contrast, perfusion parameters are calculated by deconvolution from the changes in the density–time curve for each pixel using mathematical algorithms based around the central volume principle: ,
- 1.
Mean transit time (MTT) indicates the time difference between the arterial inflow and venous outflow.
- 2.
Time to bolus peak (TTP) indicates the time from the beginning of contrast material injection to the maximum (peak) concentration of contrast material within a region of interest.
- 3.
Cerebral blood volume (CBV) indicates the volume of blood per unit of brain mass (normal range in gray matter, 4–6 mL/100 g).
- 4.
Cerebral blood flow (CBF) indicates the volume of blood flow per unit of brain mass per minute (normal range in gray matter, 50–60 mL/100 g/min). The relationship between CBF and CBV is expressed by the equation CBF = CBV/MTT.
The main advantage of perfusion-CT is its wide availability and quantitative accuracy. Its main limitation is its inability to image the whole brain, as it is limited to a 2–3 cm section of brain tissue per bolus. Now with the availability of 320-slice volumetric multimodal CT, it is possible to visualize dynamic changes in the blood flow of the entire brain.
Diffusion-weighted Magnetic Resonance Imaging and Diffusion Tensor Imaging
DWI is based on the measurement of the random (Brownian) motion of water molecules and detects the degree of mobility (or diffusibility) of water molecules within tissues. By introducing spatial magnetic field gradients, it is possible to obtain MR sequences that are sensitive to the diffusivity of water along a chosen direction, obtaining so-called DWI. From the ratio of DWI images acquired at different levels of diffusion sensitization (known as b-value), it is possible to compute a quantitative measure of mean diffusivity, known as the apparent diffusion coefficient (ADC). The ADC measures water diffusion and, therefore, often mirrors changes in the DWI signal. In areas of increased diffusion like vasogenic edema, DWI signal intensity is low, and there is an increase in ADC. In regions of restricted diffusion like cytotoxic edema, DWI signal intensity is increased, and the ADC signal decreases.
This technique is widely used in acute ischemic stroke in which reduced diffusion resulting in increased DWI signal and reduced ADC is seen before the onset of visible abnormalities on conventional MR imaging. , In the affected region, there is a temporal evolution from restricted diffusion (ie, cytotoxic edema in the acute stroke setting) to unrestricted diffusion (ie, vasogenic edema and encephalomalacia in the chronic setting). DWI is also used to study other CNS processes such as cerebral and spinal abscesses, epidermoid cysts, traumatic brain injury (TBI), , and studying brain maturation and development, especially the myelination process.
Water diffusion properties also play a role in DTI—a related imaging technique. Whereas gray matter is microstructurally compact with no directional preference, white matter bundles are arranged in a highly directional manner. For this reason, while water usually diffuses in all directions (meaning diffusion is isotropic) in the brain, water diffuses preferentially along white matter tracts rather than across them (meaning diffusion in white matter is anisotropic). A mathematical algorithm known as diffusion tensor is commonly used to model anisotropic diffusion in white matter. Fractional anisotropy (FA) maps can be created—FA index varies between 0 (representing a symmetrical anisotropic medium where there is no directionality of the diffusion, for instance in water) and 1 (representing maximum anisotropy). Also, directionally encoded color maps and three-dimensional tractography can be performed to visually display white matter tracts ( Fig. 5.4 ).
Perfusion-weighted Magnetic Resonance Imaging
While DWI is most useful for detecting irreversibly infarcted tissue, PWI may be used to identify areas of reversible ischemia as well. PWI techniques rely either on an exogenous method of achieving perfusion contrast (ie, the administration of an MR contrast agent typically gadopentate dimeglumine [Gd-DTPA, Magnevist®]) or on an endogenous method, which uses an endogenous diffusible tracer to measure CBF by applying magnetic resonance pulses to tag in-flowing water protons. , PWI is most commonly applied as bolus tracking following the intravenous administration of a bolus of gadolinium contrast. The passage of the contrast agent through the brain capillaries causes a transient loss of signal because of the susceptibility (T2*) effects of the contrast agent. A hemodynamic time-signal intensity curve is produced with subsequent calculation of MTT, TTP, CBF, and CBV perfusion maps using the same principles as those underlying perfusion CT imaging ( Fig. 5.5 ). , PWI can also be performed using T1-weighted imaging, also following an injection of gadolinium contrast. This technique requires a longer acquisition, but can measure the permeability of the blood–brain barrier.
Brain perfusion can also be assessed using another MRI technique called arterial spin labeling (ASL) ( Fig. 5.6 ). This method does not use an exogenous contrast agent, but rather uses an endogenous diffusible tracer to measure perfusion parameters by applying MR pulses to magnetically labeled in-flowing water protons. With increasing computer processing speeds and the speed of processing involved, this method is being increasingly used in clinical settings moving slowly away from the domain of being a purely research tool. This method is especially useful in patients who cannot be administered with contrast due to renal failure.
Magnetic Resonance Spectroscopy
MRS allows noninvasive, in vivo assessment of brain metabolism. The physical basis of MRS is the chemical shift effect. It actually means that nuclei located in different molecular environments sense slightly different magnitudes of magnetic field, causing them to process at different rates. MRS provides plots or spectra of signal intensity, which is proportional to concentration, versus precession rate shift with respect to a reference, expressed in parts per million (ppm). Various biologically relevant metabolites can be identified based on subtly different resonant frequencies, which are a reflection of their specific chemical environment. These metabolites reflect aspects of neuronal integrity, energy metabolism, and cell membrane proliferation or degradation. In clinical practice, five major metabolites containing hydrogen nuclei (1H-MRS) are typically evaluated ( Fig. 5.7 ): ,
- 1.
Creatine/phosphocreatine (Cr/PCr), 3.04 ppm: Creatine and phosphocreatine are involved in cellular energy metabolism and ATP production. As creatine and phosphocreatine levels tend to be relatively constant in the normal brain, they are used as a reference metabolite with the concentrations of other metabolites expressed as the ratio of the peak areas compared with the creatine peak.
- 2.
N-acetyl aspartate (NAA), 2.02 ppm: NAA is a cellular amino acid and is a neuronal marker and a measure of neuronal density and integrity. Reduced levels have been reported in a wide spectrum of conditions involving neuronal death or dysfunction, decreased neural metabolism, axonal/dendritic loss, reduced myelination. As most brain tumors are of non-neuronal origin, NAA is absent or greatly reduced, as it is with other insults to the brain such as infarction or demyelination that produce neuronal dysfunction or loss. A decrease in NAA has also been observed after head injury.
- 3.
Choline (Cho), 3.24 ppm: Cho is a composite signal of choline compounds (glycerophosphocholine [GPC], phosphocholine [PC] and a small amount of free choline) and thus reflects total brain choline stores. Cho is a constituent of the phospholipid metabolism of cell membranes and reflects membrane turnover. A Cho increase is characteristic of brain tumors due to accelerated membrane turnover in rapidly dividing cancer cells.
- 4.
Lactate (Lac), 1.33 ppm: Under normal conditions, lactate is barely detectable by MRS in the normal healthy brain. Increased lactate production occurs in disorders of energy metabolism and suggests altered energy metabolism and is consistent with cerebral ischemia.
- 5.
Glutamate and glutamine (Glx), composite peak between 2.1 and 2.5 ppm: Glutamate is an excitatory neurotransmitter that plays a role in mitochondrial metabolism. Gamma-aminobutyric acid is an important product of glutamate. Glutamine plays a role in detoxification and regulation of neurotransmitter activities. Elevated glutamate levels lead to excitotoxic cell damage. Increased glutamine synthesis occurs as a result of increased blood ammonia levels.
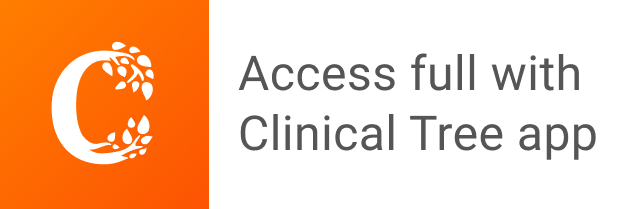