Key points
- ▪
Oxidative phosphorylation is critical to aerobic cellular energy production.
- ▪
Five enzyme complexes make up the electron transport chain, encoded by nuclear DNA (nDNA) and mitochondrial DNA (mtDNA). Mutations in mtDNA or nDNA can result in defective oxidative phosphorylation and underlie inherited mitochondrial myopathies, encephalomyopathies, and cytopathies.
- ▪
Most volatile and intravenous anesthetic agents inhibit complex I of the electron transport chain.
- ▪
Inherited mitochondrial diseases with childhood onset often present in the newborn period, with variable clinical features.
- ▪
Inherited mitochondrial diseases with adult onset can appear during the early to middle adult years, often with a decline in brain and retinal function caused by high rates of metabolic activity.
- ▪
Preoperative evaluation for patients with suspected mitochondrial myopathy includes screening (lactate/pyruvate and ketone ratios), serum and spinal fluid lactate, skeletal muscle biopsy, and assessment of glucose metabolism.
- ▪
For pediatric patients, initial investigation involves blood and urine testing, although normal lactate and glucose do not rule out mitochondrial disease. Confirmatory diagnostic studies include skin or muscle biopsies for microscopic evaluation and mtDNA analysis.
- ▪
With adult-onset mitochondrial disease, extensive preoperative assessment of organ system functional reserve is more useful than traditional tests.
- ▪
Surgical patients with mitochondrial disease are at greater risk of stroke, deteriorating neurologic status, coma, seizures, respiratory failure, arrhythmias, and death.
- ▪
Avoid long fasting times in children, and use dextrose-containing intravenous fluids. Follow malignant hyperthermia precautions, and avoid spontaneous intraoperative ventilation.
“Mitochondrial myopathy” and “inherited mitochondrial encephalomyopathy” originally encompassed a group of pediatric neurologic syndromes produced by maternally inherited mitochondrial genetic defects. However, it is now clear that respiratory chain deficiencies undermine metabolic energy production, produce excessive levels of “free radical” reactive oxygen species (ROS), and may generate almost any symptom, in any organ system, at any stage of life. Therefore, the scope of human disease attributable to the inherited, acutely acquired, or insidious onset of impaired mitochondrial function may be much broader than previously believed.
In addition to the energy production essential for life, the hundreds of mitochondria found in every cell also provide a variety of metabolic and cell regulatory functions. For example, hepatic mitochondria provide detoxification of ammonia. In neurons, mitochondria are essential for neurotransmitter synthesis. Therefore, mitochondrial dysfunction is emerging as a pivotal factor in the etiology of sepsis, neurodegenerative disorders, diabetes, arteriosclerotic disease, and even normal human aging. This chapter provides an overview of the perioperative assessment and anesthetic management of patients with inherited mitochondrial disorders of either childhood or adult onset. The effects of common anesthetic agents on mitochondrial function are discussed, with a review of the mitochondrial basis of anesthesia-induced neurotoxicity in the developing brain.
Background
Mitochondria produce adenosine triphosphate (ATP) by oxidative phosphorylation through the electron transport chain, which is composed of five enzyme complexes located on the inner mitochondrial membrane ( Fig. 14-1 ). Reduction of molecular oxygen is coupled to phosphorylation of adenosine diphosphate (ADP), resulting in ATP synthesis. The reduced cofactors NADH and FADH 2 , generated by the Krebs cycle and by fatty acid oxidation, donate electrons to complex I (NADH dehydrogenase) and complex II (succinate dehydrogenase). Electrons are then transferred to coenzyme Q and subsequently to complex III. From complex III, reduced cytochrome c donates its electrons to complex IV (cytochrome- c oxidase), resulting in the reduction of molecular oxygen to water. Complexes I, III, and IV actively pump hydrogen ions (H + ) across the inner membrane of the mitochondrion into the intermembrane space, creating an electrochemical gradient. Influx of protons back into the mitochondrial matrix through complex V results in ATP synthesis. This process of oxidative phosphorylation is the major intracellular source of the free radicals (O 2 − , H 2 O 2 , and OH − ) that are generated as byproducts of the interaction between excess electrons and oxygen.

The enzymes, membranes, and other molecular components of these five major enzyme/protein complexes needed for mitochondrial oxidative phosphorylation are encoded in a complementary manner by the circular genome found within the mitochondrion itself, as well as by the much larger nuclear genome of the host cell. The mitochondrial genome encodes for 13 essential subunits of the electron transport chain, two types of ribosomal ribonucleic acid (rRNA), and 22 forms of transfer RNA (tRNA). Each mitochondrion contains multiple copies of mitochondrial deoxyribonucleic acid (mtDNA). Nuclear DNA (nDNA) encodes an additional 900 proteins that are needed for normal mitochondrial function.
The complementary relationship between two genomes within each cell and the putative evolution of the mitochondrion from a free-living organism into an organelle within the cell have been known and discussed by cell biologists only within the past three or four decades. The implications of this biologic curiosity with regard to our understanding of embryology, evolution, aging, and even the mechanism of death itself may be profound. The mitochondrion, through a central role in the modulation of bioenergetics and cellular apoptosis, may also serve as both a “biosensor” for oxidative stress and as the final determinant of cellular viability.
The most severe inherited mitochondrial disease syndromes become clinically apparent during infancy, but a few were eventually described in which symptoms did not appear until early adulthood. The original descriptions of the mitochondrial diseases of childhood assumed that there was maternal transmission of mitochondria and of both normal (“wild type”) and mutant mtDNA. Because mutant mtDNA coexists with wild-type mtDNA, variability in the severity of all these inherited conditions is thought to reflect heteroplasmy, the random differences in the proportion of mutant mtDNA distributed throughout the target tissues during embryogenesis. For the mitochondrial disorders of adult onset, variability in disease severity and an exceptionally wide range of phenotypic symptom patterns are thought to reflect both heteroplasmy and the much different, progressively changing metabolic demands of target tissues during adulthood. Hundreds of mtDNA mutations have already been identified in detail and classified as mitochondrial myopathies, encephalomyopathies, or cytopathies.
A report of a patient with mutated mtDNA of paternal origin, however, suggests that some paternal mtDNA also survives in the zygote and therefore may also contribute to the mtDNA pool. Recently, the important role of defects in nDNA in disorders characterized by declining mitochondrial bioenergetics has also been clarified, reflecting a better understanding of the interaction between nuclear and mitochondrial genomes. It is now clear that there are subunits of the electron transport chain not encoded by mtDNA that arise from nDNA. Diseases caused by nuclear genes that do not encode subunits but affect mtDNA stability are an especially interesting group of mitochondrial disorders. In these syndromes, a primary nuclear gene defect causes secondary mtDNA information loss or deletion, which leads to subsequent tissue dysfunction in the form of disrupted oxidative phosphorylation. Therefore, some genetically determined defects in oxidative phosphorylation follow classic mendelian patterns of dominant recessive genetic transmission rather than the maternal patterns usually associated with mtDNA defects.
Effect of anesthetics on mitochondrial function
The effects of anesthetics on mitochondrial function were first investigated in the 1930s. Although all the mechanisms of action are still not established, virtually all volatile, local, and intravenous (IV) anesthetics clearly have significant depressant effects on mitochondrial energy production. These effects are believed to occur primarily at the level of the electron transport chain on the inner membrane of mitochondria. Early studies reported inhibition of the oxidation of glucose, lactate, and pyruvate by narcotics, and more recent work explores the mechanism of reduced oxygen consumption in the brain after treatment with barbiturates. A common final pathway of depressed bioenergetic activity, possibly through intracellular or mitochondrial mechanisms, may also help explain the primary anesthetic effects of these drugs.
However, these data must be interpreted cautiously, because much of the work examining anesthetic-induced mitochondrial dysfunction has been done in vitro, in isolated mitochondria, and not in functioning cells. Furthermore, the anesthetic concentrations used to inhibit mitochondrial function experimentally have been up to 10-fold higher than concentrations used clinically, although anesthetics seem to inhibit mitochondria in a dose-dependent manner. The reader must take these major limitations into consideration when reviewing the subject.
Inhalational and Local Anesthetics
Nitrous oxide (N 2 O) and the potent inhalational agents have significant effects on mitochondrial respiration. In cardiac mitochondria, halothane, isoflurane, and sevoflurane have all been shown to inhibit complex I of the electron transport chain. At concentrations equal to 2 MAC (minimal alveolar concentration), complex I activity is reduced by 20% after exposure to halothane and isoflurane, and by 10% after exposure to sevoflurane. Oxidative phosphorylation in liver mitochondria is also measurably disrupted after exposure to halothane. Concentrations of 0.5% to 2% halothane lead to reversible inhibition of complex I (NADH: ubiquinone oxidoreductase). Halothane-induced mitochondrial inhibition in the liver is exacerbated by the addition of N 2 O. Local anesthetics also disrupt oxidative phosphorylation and significantly degrade bioenergetic capacity in mitochondrial isolates.
Barbiturates, Propofol, Etomidate, Ketamine, Midazolam
Barbiturate effects have been well studied in the brain, heart, and liver. As with the inhalational agents, barbiturates inhibit complex I of the electron transport chain. This inhibition, however, occurs at serum levels that greatly exceed those required to produce the anesthetic effect. Propofol disrupts electron transport in the respiratory chain. Decreased oxygen consumption and inhibited electron flow have been demonstrated in cardiac mitochondria exposed to propofol. Similarly, work with mitochondria from the liver has demonstrated that propofol inhibits complex I of the electron transport chain. Etomidate has also been shown to inhibit oxidative phosphorylation in isolated rat hepatic mitochondria. The inhibitory effect of etomidate occurs mostly at the level of complex I and to a lesser extent at complex III. In addition, low concentrations of ketamine inhibit the NAD + -linked oxidation of glutamate and malate in rat hepatic mitochondria, but can also uncouple oxidative phosphorylation. Midazolam, a common benzodiazepine, inhibits complexes I, II, and III. Box 14-1 summarizes the effect of various anesthetic agents on mitochondrial function.
Volatile agents inhibit complex I.
Barbiturates inhibit complex I.
Propofol inhibits complex I and slows electron transport chain.
Etomidate inhibits complex I and complex III.
Ketamine inhibits complex I.
Midazolam inhibits complexes I, II, and III.
Local anesthetics disrupt oxidative phosphorylation by unknown mechanisms.
Other Effects
Exposure to the volatile anesthetics also alters the ability of the mitochondrion to respond to rising levels of ROS, a “preconditioning” effect that may protect the cell if exposed later to periods of hypoxia or ischemia. Although the mechanism of “anesthetic preconditioning” remains speculative, anesthetic agents appear to disrupt mitochondrial bioenergetics sufficiently that they produce the low levels of oxidative stress that induce short-term genetic expression of heat shock protein (HSP) or other protective substances. HSP can also be induced by brief, sublethal episodes of ischemia or hypoxia, suggesting that the prophylactic administration of HSP or similar interventions may provide cardiac and neural protection during major surgery when tissue perfusion or oxygenation is disrupted.
Therefore, anesthetics may not only depress bioenergetic activity, but also affect other functions of the mitochondrion, such as the role of this organelle as a “biosensor” for oxidative stress, or the mitochondrion as an effector organelle for cellular apoptosis. Accumulation of ROS increases outer-membrane permeability of the mitochondrion and leads to the ingress of potassium and ionized calcium and the release of cytochrome c and other “pro-apoptotic” soluble proteins. Release of cytochrome c from mitochondria not only rapidly degrades the bioenergetic capacity of the cell by removing a key component of the respiratory chain, but also appears to trigger the release of caspases, cysteine-containing protease enzymes. Caspases in turn activate other enzymes that digest nDNA, the final step in “cell suicide,” or apoptosis.
Anesthesia-Induced Neurotoxicity
Recent studies show that common anesthetics (benzodiazepines, barbiturates, ketamine, inhaled volatile anesthetics, N 2 O) induce widespread neuronal apoptosis in the developing brain in a variety of experimental newborn animal models (rodents, guinea pigs, piglets, primates) raising serious concern among anesthesiologists. Although anesthesia-induced neurotoxicity has never been demonstrated in human infants, two studies suggest a potential association between anesthesia exposure and developmental and behavioral disorders in younger children. In the first study, almost 600 children were retrospectively compared to 4764 controls. Those who received two or more general anesthetics before age 4 years were at increased risk for having a learning disability. In another retrospective analysis, 383 children who underwent inguinal hernia repair under general anesthesia within the first 3 years of life were compared to 5050 age-matched controls. The anesthesia-exposed cohort was more than twice as likely to have a subsequent diagnosis of developmental or behavioral disorder than controls. Although no causative relationship was established, these studies question the safety of anesthesia exposure during infancy and childhood.
The mechanism of anesthesia-induced neurotoxicity appears to be caspase activation through the mitochondrial pathway of apoptosis, followed by activation of the “death receptor pathway” and neurotrophic factor–activated pathways ( Fig. 14-2 ). This results in massive caspase-3 activation, subsequent nDNA fragmentation, and cell death. Although chemically dissimilar, many different anesthetic agents inhibit neuronal activity and synaptic transmission through interaction with γ-aminobutyric acid (GABA) and N- methyl- d -aspartate (NMDA) receptors in the brain. After this GABA and NMDA receptor interaction, Bax protein translocates to mitochondria, causing release of cytochrome c from the mitochondria intermembrane space into the cytosol. Cytochrome c then complexes with apoptotic protease activation factor 1 (APAF-1) and deoxyadenosine triphosphate dATP. This complex then oligomerizes and recruits and activates procaspase-9. Subsequent procaspase 3 recruitment forms the apoptosome and activates caspase 3. This results in nDNA fragmentation.

All the experimental data to date indicate that the vulnerability of the newborn mammalian brain to anesthesia-induced neurotoxicity coincides with synaptogenesis. Furthermore, anesthesia-induced neuroapoptosis has resulted in significant neuronal loss, behavioral impairments, and cognitive deficits. Whether such neurotoxicity occurs in the human system is unknown, however, so future investigations need to focus on identifying evidence of anesthesia-induced neuroapoptosis in human infants and assess neurodevelopmental outcome after anesthetic exposure during infancy and childhood.
Inherited disorders with childhood onset
Mitochondrial diseases with childhood onset often present in the newborn period. The clinical features may vary because a single organ system or multiple organ systems may be affected. The organ systems most often involved are the central nervous system (CNS), peripheral nervous system (PNS), liver, heart, kidneys, muscle, gastrointestinal (GI) tract, the skin, and a number of endocrine glands. Nonspecific signs include lethargy, irritability, hyperactivity, and poor feeding. The presentation can be very abrupt and dramatic, with acute onset of hypothermia or hyperthermia, cyanosis, seizures, emesis, diarrhea, or jaundice. Box 14-2 lists some of the more insidious signs and symptoms of mitochondrial disease in the newborn.
Unexplained sepsis or recurrent severe infection
Unexplained hypotonia, weakness, failure to thrive, and metabolic acidosis
Organic acidemias such as maple syrup urine disease and methylmalonic aciduria
Urea cycle defects such as ornithine transcarbamylase deficiency
Carbohydrate disorders such as galactosemia and hereditary fructose intolerance
Aminoacidopathies such as homocystinuria, tyrosinemia, and nonketotic hyperglycemia
Endocrinopathies such as congenital adrenal hyperplasia and congenital diabetes
The mtDNA depletion syndrome (MDS) is a severe disease of childhood characterized by liver failure and neurologic abnormalities involving tissue-specific loss of mtDNA. MDS is thought to be caused by a putative nuclear gene that controls mtDNA replication or stability. Similarly, children with mitochondrial neurogastrointestinal encephalomyopathy (MNGIE) may have multiple mtDNA deletions and mtDNA depletion resulting from an nDNA mutation. Although found in most mitochondrial disorders, nonspecific GI and hepatic symptoms are among the cardinal manifestations of primary mitochondrial diseases such as MDS and MNGIE.
The CNS manifestations of mitochondrial disorders include encephalopathy, a cardinal feature of Leigh’s syndrome. Seizures and ataxia also occur with myoclonic epilepsy with ragged-red fibers (MERRF). Dementia and stroke-like symptoms are major features of mitochondrial encephalomyopathy with lactic acidosis and stroke-like episodes (MELAS). When the PNS is involved, there may be axonal sensory neuropathies. Cardiac involvement with pediatric mitochondrial disease may produce hypertrophic cardiomyopathy, seen with MELAS, or dilated cardiomyopathy, heart block, and pre-excitation syndrome, features of Leber’s hereditary optic neuropathy (LHON). Impaired renal bioenergetics produces tubular acidosis; muscle abnormalities present largely as myopathies. Hepatic failure, dysphagia, pseudo-obstruction, and constipation all suggest GI impairment. Vision and hearing are often impaired by ophthalmoplegia, ptosis, cataracts, optic atrophy, pigmentary retinopathy, and sensorineural deafness. Endocrine organ involvement manifests with diabetes mellitus, hypoparathyroidism, hypothyroidism, and gonadal failure.
Table 14-1 lists typical symptoms and signs of the most well-known mtDNA-related syndromes.
Disease | Mutation | Inheritance | Signs and Symptoms |
---|---|---|---|
Kearns-Sayre syndrome | Large-scale mtDNA deletion | Sporadic | Ataxia, peripheral neuropathy, muscle weakness, ophthalmoplegia, ptosis, pigmentary retinopathy, sideroblastic anemia, diabetes mellitus, short stature, hypoparathyroidism, cardiomyopathy, conduction defects, sensorineural hearing loss, Fanconi syndrome, lactic acidosis, ragged-red fibers on muscle biopsy |
Progressive external ophthalmoplegia | Large-scale mtDNA deletion | Sporadic | Muscle weakness, ophthalmoplegia, ptosis, lactic acidosis, ragged-red fibers on muscle biopsy |
Pearson’s syndrome | Large-scale mtDNA deletion | Sporadic | Ophthalmoplegia, sideroblastic anemia, pancreatic dysfunction, Fanconi syndrome, lactic acidosis, ragged-red fibers on muscle biopsy |
Myoclonic epilepsy with ragged-red fibers (MERRF) | mtDNA point mutation, tRNA abnormality | Maternal | Seizures, ataxia, myoclonus, psychomotor regression, peripheral neuropathy, muscle weakness, short stature, sensorineural hearing loss, lactic acidosis, ragged-red fibers on muscle biopsy |
Mitochondrial encephalopathy with lactic acidosis and stroke-like episodes (MELAS) | mtDNA point mutation, tRNA abnormality | Maternal | Seizures, ataxia, myoclonus, psychomotor regression, hemiparesis, cortical blindness, migraine, dystonia, peripheral neuropathy, muscle weakness, diabetes mellitus, short stature, cardiomyopathy, conduction defects, intestinal pseudo-obstruction, sensorineural hearing loss, Fanconi syndrome, lactic acidosis, ragged-red fibers on muscle biopsy |
Aminoglycoside-induced deafness | tRNA abnormality | — | Cardiomyopathy, sensorineural hearing loss |
Neuropathy, ataxia, and retinitis pigmentosa (NARP) | mtDNA point mutations, mRNA abnormality | Maternal | Ataxia, peripheral neuropathy, muscle weakness, pigmentary retinopathy, optic atrophy, sensorineural hearing loss |
Maternally inherited Leigh’s syndrome | mtDNA point mutation, mRNA abnormality | Maternal | Seizures, ataxia, psychomotor regression, dystonia, muscle weakness, pigmentary retinopathy, optic atrophy, cardiomyopathy, lactic acidosis |
Leber’s hereditary optic neuropathy (LHON) | Multiple mtDNA point mutations, mRNA abnormality | Maternal | Dystonia, optic atrophy, conduction defects |
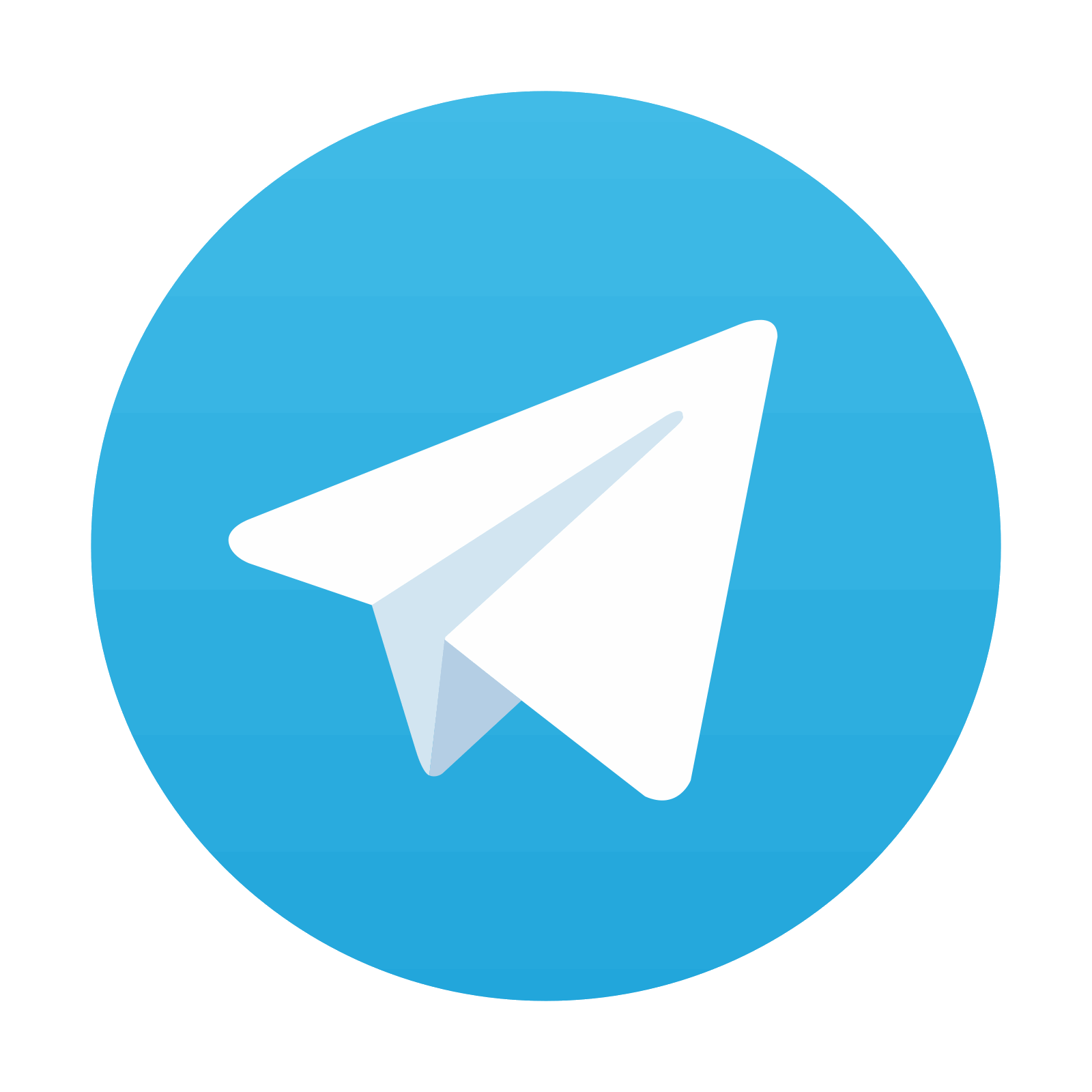
Stay updated, free articles. Join our Telegram channel
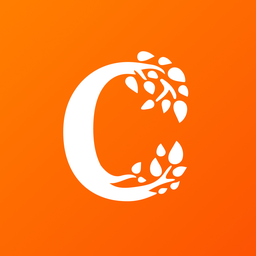
Full access? Get Clinical Tree
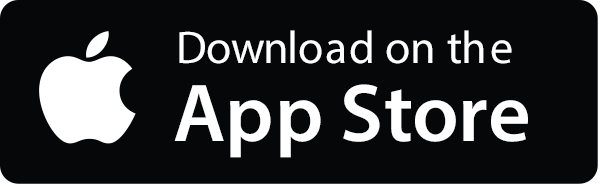
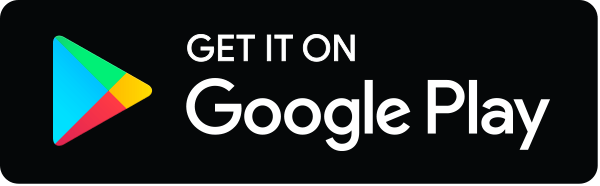
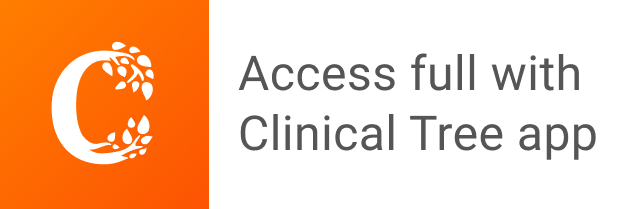