where Lp represents hydraulic permeability (fluid conductivity), S is the surface area available for fluid exchange, reflecting the number of perfused capillaries, ΔP is the net transcapillary hydrostatic force for filtration, σ is the reflection coefficient for macromolecules (plasma proteins), and Δπ is the transcapillary oncotic absorbing pressure force.
The reflection coefficient for plasma proteins describes the effective part of the transcapillary oncotic pressure counteracting fluid filtration, and represents the difficulty (relative to water) with which the proteins pass the exchange vessels. The reflection coefficient is 1.0 when the membrane is impermeable to the molecules and 0 when the molecules pass through the membrane without any hindrance.
The reflection coefficient for proteins is below 1.0 in all organs of the body except the brain. For albumin it is 0.90–0.95 in skeletal muscle, 0.50–0.65 in the lung, and 0.8 in the intestine and in the subcutis. A reflection coefficient below 1.0 means a continuous leakage of proteins to the interstitium. A system with recirculation of proteins between blood vessels and tissue and back to the intravascular space is essential to allow access of antibodies, protein-bound hormones, cytokines, and other macromolecules to the interstitial space. The reflection coefficient can be reduced significantly under a state of inflammation, leading to increased loss of albumin and other macromolecules to the interstitium. Tissue edema is formed when the leakage of plasma fluid is greater than the net recirculating capacity involving the lymphatic system, and hypovolemia develops in parallel.
The Starling formula, however, gives no information about the mechanisms responsible for the protein leakage. There has been a general belief for decades that active (energy-consuming) transcytosis (vesicle transport) across the endothelial membrane of the capillaries is responsible for a large part of the protein loss to the interstitium.[2] However, it is unlikely that the relatively small capacity of an active vesicle transporting mechanism would be responsible for transport of as much as 5–6% per hour of all intravascular plasma proteins – and even more than that under inflammatory states. The capacity of an energy-consuming process is also reduced by inflammation. Furthermore, blockade of transcytosis by cooling or pharmacological inhibition in the rat was not found to reduce TER.[3,4] Therefore, transport of proteins across the capillary membrane should be mainly a passive process independent of energy-consuming activities, as discussed below.
The two-pore theory
By the alternative two-pore theory for transcapillary fluid and protein exchange,[5] the large loss of proteins from the intravascular to the extravascular space can be explained by passive mechanisms. According to this theory, fluid and small solutes pass the capillary membrane through all the pores along the entire capillary bed and in venules, whereas proteins pass the membrane mainly through the much less abundant (by a factor of 10–30 thousand) large pores on the venous side of the capillary network, and in the venules (Figure 10.1).
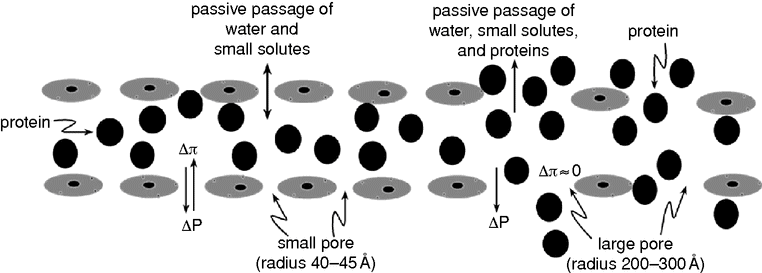
A schematic illustration of the principles used in control of transvascular exchange of fluid and macromolecules according to the two-pore theory. Absence of an oncotic absorbing force across the large pore means that the hydrostatic transcapillary force (ΔP) is the main force creating a “jet” stream of protein-rich fluid through each large pore, mainly via convection from the intravascular to the extravascular space. An increase in the number and the area of large pores and an increase in the hydrostatic transcapillary pressure will increase the loss of proteins.
Whereas the small pores with their radius of 40–45 Å are freely permeable to electrolytes and other small molecules and much less permeable to proteins, the large pores with their radius of about 250 Å are also freely permeable to proteins. This means that the oncotic pressure gradient across the large pore is low. The protein loss to the interstitium via the large pores can partly be explained by diffusion, but the main mechanism is convection when the proteins follow the large-pore fluid stream with the transcapillary/transvenular hydrostatic pressure as the major driving force. This means that the protein loss depends on both the number and size of large pores (large-pore permeability) and the transcapillary/transvenular hydrostatic pressure.
The lymphatic system
The capacity of the lymphatic system is of great importance for maintenance of a balance between the intravascular and the interstitial space. A normal TER of 5–6% of plasma volume per hour, corresponding to 150–200 ml plasma per hour for an adult, is in the range of the capacity of the lymphatic system under normal circumstances.[1] The capacity of the lymphatic drainage increases with increase in skeletal muscle activity and it can be reduced by inactivity, such as in immobile patients.
If TER is increased, such as after trauma and during sepsis/SIRS, the capacity of the lymphatic system may be too low to transfer enough leaking plasma fluid back to the circulation, and tissue edema in combination with hypovolemia develops. The situation will be aggravated in immobile patients by the reduced recirculating capacity of the lymphatic system.
Physiotherapy might improve this capacity and help to counteract tissue edema, hypovolemia, and the need for plasma volume expanders in critically ill patients.
Transvascular fluid exchange in the brain
The volume regulation of the brain is more effective than that of other organs of the body. This is of importance as there is no room for expansion in volume of the brain when it is tightly enclosed in the cranium. The brain also lacks a lymphatic drainage system.[6] The sophisticated regulation of the brain volume can be ascribed to the low permeability of cerebral capillaries, which creates the so-called blood–brain barrier (BBB). The cerebrospinal fluid system may also be involved to maintain a normal intracranial pressure.
The intact BBB means that the cerebral capillaries are impermeable to passive transport of all molecules except water. Thus, neither macromolecules such as proteins nor small solutes such as sodium or chloride ions can pass the cerebral capillary passively. This means that a disturbed balance between the hydrostatic and the oncotic pressures will result in transfer of only water across the cerebral capillaries, and the subsequent dilution/concentration of the crystalloid osmotic pressure of brain interstitium will result in immediate interruption of further fluid exchange.[6]
In the injured brain with a disrupted BBB – in the sense that it has also become passively permeable for small solutes – there will be much less dilution during filtration. The filtration will continue for a longer time, creating a vasogenic brain edema, and it will finally be balanced by the increase in intracranial pressure (ICP).[7]
Crystalloids and colloids as plasma volume expanders
While the healthy patient is normovolemic and needs no extra plasma volume expansion, most critically ill patients develop hypovolemia due to increased leakage of plasma fluid that exceeds the capacity of the absorbing effect and the lymphatic system. However, there is still debate regarding which plasma volume expander should be used to maintain or achieve normovolemia. In this chapter we attempt to describe the advantages and drawbacks of using crystalloids and various colloids as plasma volume expanders.
Because of the small radius of electrolytes (<2–3 Å) relative to the radius of the small pores (40–45 Å), the reflection coefficient of electrolytes is very small. The infused crystalloid solution is therefore distributed relatively quickly over the whole extracellular space, and more or less independently of the prevailing microvascular permeability, also resulting in reduction in plasma and interstitial oncotic pressure. As plasma volume is only about 20–25% of total extracellular volume, only 20–25% of the infused solution can be used for plasma volume expansion and the rest will result in tissue edema. A simultaneous increase in urine production must be compensated by further infusions to maintain normovolemia.
Tissue edema not only has cosmetic consequences, but it can also be a real drawback in terms of pulmonary edema, acute respiratory distress syndrome (ARDS), and increased intercapillary distances. It may also compromise perfusion because of an increased tissue pressure, and there is a risk of compartment syndrome. Reduction in plasma oncotic pressure may also result in further filtration according to the Starling formula. Distribution of a crystalloid solution to the interstitium will not occur in the normal brain because of the intact BBB, but it may occur to some extent in the injured brain if the cerebral capillaries have become permeable to small solutes, aggravating a vasogenic brain edema.[7,8] Crystalloid solutions with high concentrations of chloride ions may cause hyperchloremic acidosis. This can be avoided by using balanced crystalloid solutions instead.
Albumin is the predominant protein in plasma and the only natural colloid solution recommended for plasma volume expansion today, as transfusion with plasma is justified mainly to compensate for coagulation disturbances. The albumin molecule is monodisperse with a molecular weight of 69 kDa and the solutions are available in various concentrations (3.5%, 4%, 5%, 20%, and 25%, preferably in an isotonic solution). The albumin molecule is negatively charged, and this may moderate its distribution to the interstitium. It is transported back to the intravascular compartment via the lymphatic system. In contrast to synthetic colloids, it is degraded very slowly, which can be of advantage by acting as a plasma volume expander for a longer time; but this can also be a disadvantage when it accumulates in the interstitium. The effectiveness of albumin as a plasma volume expander has been questioned, however, as some studies could not demonstrate better outcome with albumin than with saline.[9] It is suggested that the beneficial absorbing effect of albumin has been overestimated, especially during states of inflammation. At a general increase in permeability, more plasma fluid and proteins will leak to the interstitium, and the effectiveness of albumin as a plasma volume expander will be reduced. Further, a revision of the classical Starling principles incorporating the endothelial glycocalyx layer means reduced absorption effect of transcapillary oncotic pressure in favor of the hydrostatic capillary pressure.[10] This hypothesis, however, is highly controversial and has still not been confirmed.[11] These mechanisms taken together may explain why the plasma expanding effect of albumin is not as good as expected. The effectiveness of albumin as a plasma volume expander can be improved and side effects reduced by adhering to specific rules in its administration, as will be described below. Allergic reactions are rare.
The synthetic colloids available today are dextran, hydroxyethyl starch (HES), and gelatin – and they are all cheaper than albumin. In contrast to albumin, which is monodisperse, they are all polydisperse with molecular weights ranging from low to higher values, and with the majority of molecules concentrated in the range of the average molecular weight. They are degraded in plasma and in the interstitium. Their recirculation back to the intravascular space via the lymphatic system is less effective than for albumin. When degraded to molecules with weights below their renal threshold, they are also lost via the kidneys.
Dextran solutions are produced by hydroxylation of polysaccharides. The predominant dextran solution is dextran 70, with an average molecular weight of 70 kDa. It is slowly degraded to CO2 and water or lost via the kidneys. It improves the microcirculation and has the most effective and long-lasting plasma volume-expanding effect of all colloids available for clinical use today.[12,13] The risk of anaphylaxis is very low on pretreatment with 1 kDa dextran molecules (hapten competitor dextran 1), and therefore this should be obligatory. By its influence on platelet function, dextran can exacerbate an underlying coagulopathy, which limits its use in larger volumes during surgery or other situations with risk of bleeding. In larger volumes, the beneficial effect on microcirculation, therefore, should be weighed against the risk of increased tendency of bleeding. It is of great value preferentially in intensive care through its good and long-lasting plasma volume-expanding effect, through improving microcirculation, and through its prophylactic effect against thrombosis.
Owing to side effects with HES solutions of higher molecular weights, the third generation of HES solutions – with mean molecular weight of 130 kDa and molar substitution of approximately 0.4 – now predominate. The HES molecules are degraded relatively rapidly by amylase to smaller molecules below the renal threshold and cleared from the circulation via the kidney. The half-time of the plasma concentration of HES130 after a bolus infusion is 1.5 to 2 hours, and it is almost totally eliminated after 4 hours.[14] This has made the third generation of HES solutions less effective as plasma volume expanders, but this can be compensated for by repeating the infusion. Allergic reactions to the modern HES solutions are less common. The coagulopathic effect appears to be smaller with HES than with dextran. The use of HES solutions as plasma volume expanders to septic patients, however, was recently questioned after publication of a multi-centre study, showing increased frequency of renal failure and increased mortality in septic patients given HES.[15]
Gelatin solutions are derived from bovine collagen. The mean molecular weight of molecules in gelatin solutions is only 35 kDa, which means that it is on the borderline to be defined as a crystalloid solution. This fact in combination with the relatively fast degradation of gelatin molecules and loss via the kidneys explains their relatively poor and short-lasting plasma volume-expanding effect. Gelatin has no or only moderate effects on coagulation, but anaphylactic reactions are more common with gelatin than with other synthetic colloids.
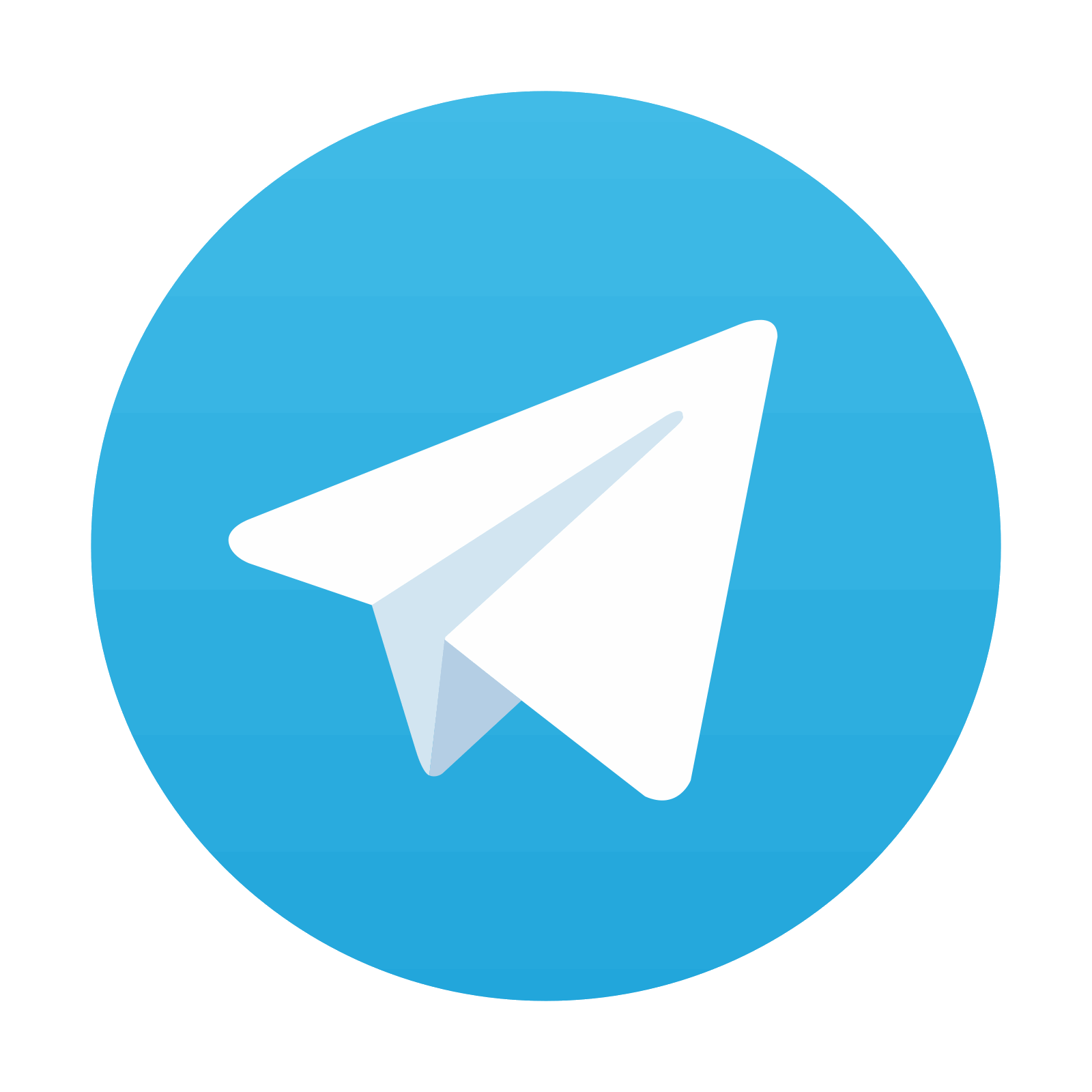
Stay updated, free articles. Join our Telegram channel
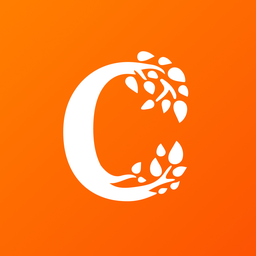
Full access? Get Clinical Tree
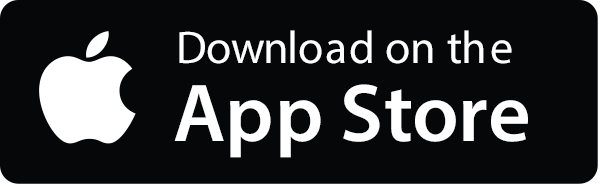
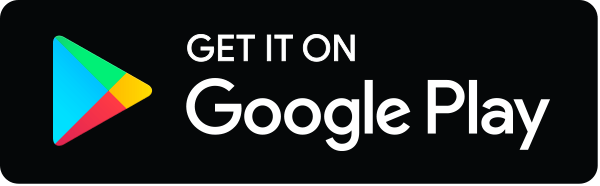