


neurons. The downregulation of SNAT5 can result in a decrease in excitatory neurotransmission in the brain and lead to excessive neuroinhibition, which is characteristic of encephalopathy in hyperammonemia (5,6,7,8). Glutamine is also thought to disrupt mitochondrial permeability by interference with the mitochondria-permeability-transition process and liberation of free radicals through hydrolysis of ammonia, all subsequently leading to mitochondrial swelling and dysfunction (9). Another hypothesis for hyperammonemia-induced encephalopathy is injury through the effect of increased production of glutamine that leads to an excess of extracellular glutamate, which induces neuronal hyperexcitability through activation of a subgroup of the glutamate family of receptors, the N-methyl-D-aspartate (NMDA) ionotropic receptors. Activation of NMDA receptors leads to depolarization and downstream changes in nitric oxide (NO) metabolism, disturbances in sodium/potassium adenosine triphosphatase (Na+/K+-ATPase) function, mitochondrial dysfunction, energy failure, and oxidative stress, together causing cell death (10). Finally, a high level of ammonia has the potential for participating in causing brain injury and cerebral edema by inducing hyperemia in cerebral blood flow (CBF) and by impairing cerebral autoregulation (11,12).
TABLE 66.1 CAUSES OF METABOLIC ENCEPHALOPATHIES CLASSIFIED ACCORDING TO PATHOPHYSIOLOGY | ||||||||||||||||||
---|---|---|---|---|---|---|---|---|---|---|---|---|---|---|---|---|---|---|
|
![]() FIGURE 66.2. Schematic representation of a glutamate/glutamine cycle. The figure illustrates position of the astrocytic glutamine synthetase (GS), neuronal glutaminase (GLNase), the astrocytic glutamate transporter EAAT-2, and the astrocyte and neuron. (From Desjardins P, Du T, Jiang W, et al. Pathogenesis of hepatic encephalopathy and brain edema in acute liver failure: Role of glutamine redefined. Neurochem Int 2012;60(7):690-6, with permission.) Gln, glutamine; Glu, glutamate; NH3, ammonia; CSF, cerebral spinal fluid. |

ligand levels in patients with hepatic encephalopathy, was first considered a possibility when similarities between evoked potential in rats with liver failure and animals treated with GABA receptor agonists were noted (13). Animal studies in models of hepatic encephalopathy have also demonstrated a beneficial effect on arousal of administering flumazenil, a GABA receptor antagonist. A few studies in humans have shown the same benefit (20), but the origin of increased GABA receptor agonists in hepatic encephalopathy is unclear; it is possible that the agonist or a precursor is contained within the food cycle, is synthesized by gastrointestinal flora, or that there is occult ingestion of pharmaceutical benzodiazepine (21,22). It is also possible that endogenous levels of the neurosteroid hormone dehydroepiandrosterone sulfate, found to be low in humans with cirrhosis, may contribute to increased GABAergic tone. Improvement in encephalopathy after administering flumazenil supports this hypothesis. However, the improvement is transient and incomplete, which demonstrates the importance of other toxic mechanisms in the pathogenesis of hepatic encephalopathy, such as glutamine-induced astrocytic swelling (see above).
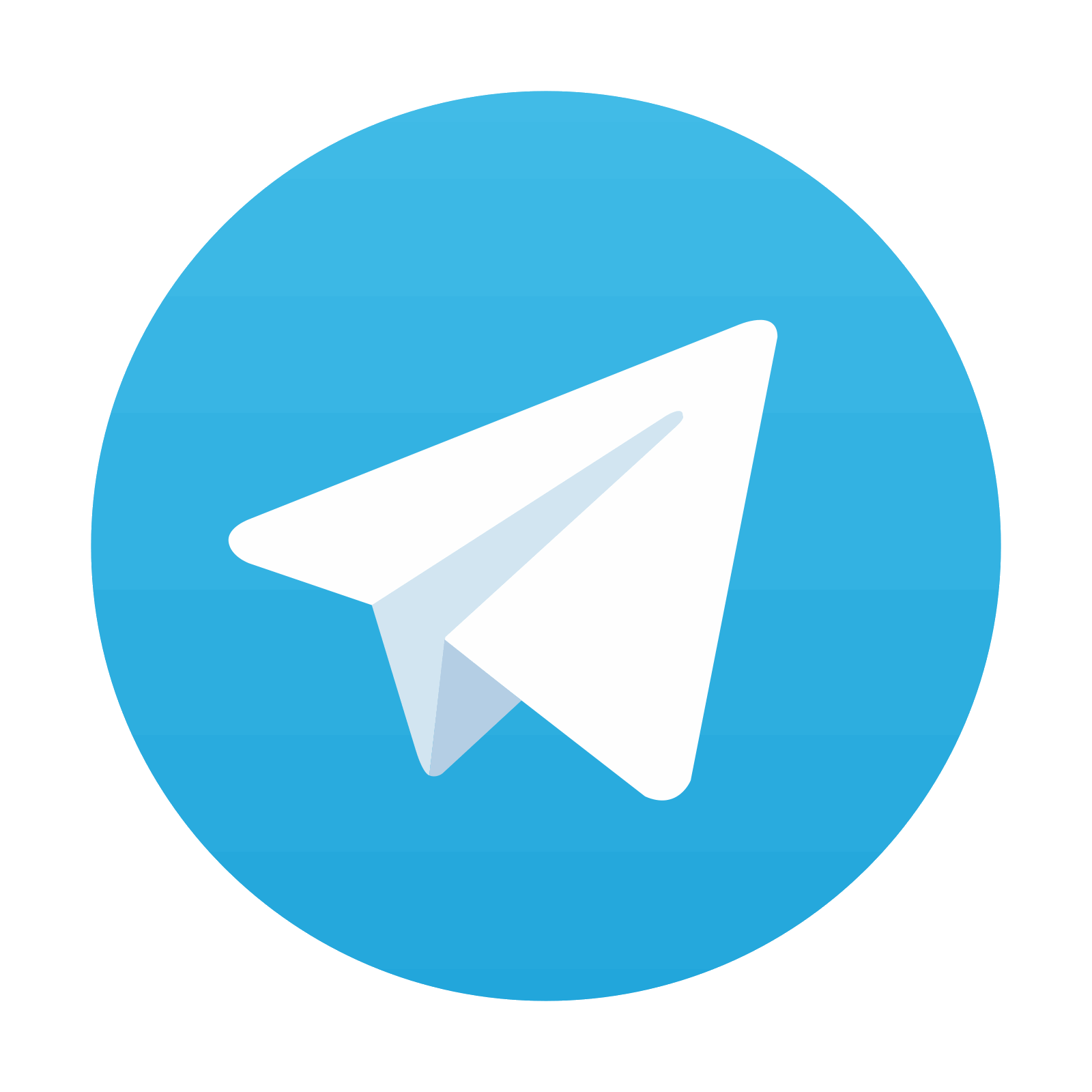
Stay updated, free articles. Join our Telegram channel
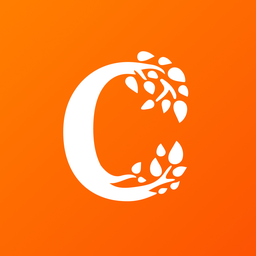
Full access? Get Clinical Tree
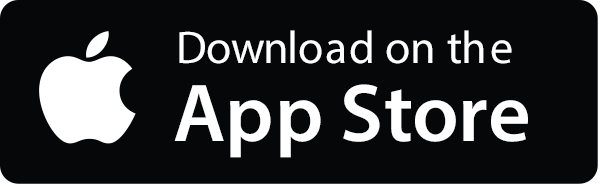
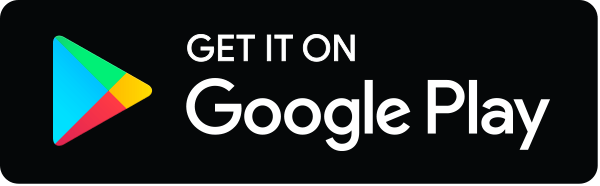
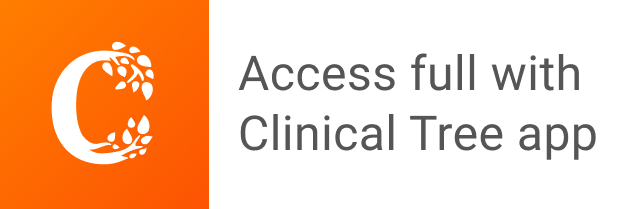