Abnormal vessel walla
Endothelial dysfunction characterized by endothelial cell activation (due to hypoxia, distension, and neurohormonal activation), increased expression of platelet/monocyte adhesion, tissue factor, von Willebrand factor, and thrombin; decreased expression of nitric oxide, thrombomodulin (due to increase of tumor necrosis factor-α and interleukin-1) and protein C
Abnormal blood flowa
Arterial vessels: abnormalities of hemorheology and turbulence at bifurcations and stenotic regions, altered high shear stress and platelet activation. Venous vessels: reduced blood flow secondary to immobility, hypoxia, and reduced clearance of activated coagulation factors
Abnormal blood constituentsa
Ruptured plaque or endothelium injury induces exposure of prothrombotic factors, enhanced platelet reactivity, increasing expression of fibrinogen and von Willebrand factor. Stasis could induce systemic and local hypercoagulability, tissue factor-bearing microparticles. Endogenous fibrinolysis hypoactivity and metabolic factors as lipoprotein (a) and hyperglycemia
Another interesting metabolic factor linking with thrombosis is the serum glucose. Elevated levels have a procoagulant effect and decrease fibrinolysis. Hyperglycemia is often accompanied by hyperinsulinemia, which may further inhibit fibrinolysis and increase the prothrombotic effect of hyperglycemia. In addition, it is possible that hyperglycemia acts as marker of increased stress and severity of illness. In acute illnesses, such as PE, stress hormones (i.e., catecholamine, growth hormone, cortisol, and cytokines) are released with increasing hepatic glucose production and insulin resistance [30]. Lipoprotein (a) and hyperglycemia could be the lost link among metabolic and thrombosis disorders.
In Virchow’s triad of the twenty-first century, endothelial dysfunction emerges as the most important component. Endothelial dysfunction can be regarded as the key of the risk factors and a syndrome that exhibits systemic manifestations associated with significant morbidity and mortality [18]. As part of endothelial function, endogenous fibrinolysis is a protective mechanism against lasting arterial or venous thrombotic occlusion, which would otherwise lead to permanent tissue damage. Because thrombogenesis is an active, ongoing, and dynamic process, a healthy endogenous fibrinolytic system can prevent the build-up of thrombus before complete occlusion occurs or before breakup of the occlusive thrombus (myocardial infarction, stroke, and limb ischemia or VTE), thus preventing tissue damage [19].
Inflammation
A growing body of evidence links inflammation with an important pathogenic role in several cardiovascular diseases related to thrombosis [4, 5, 31]. Evidence accumulated over the past two decades supports a key role for inflammation in all phases of the atherosclerotic process [10], from endothelial dysfunction and plaque formation through its progression and ultimately to the thrombotic complications that lead to the acute coronary syndrome [4]. Activated inflammatory cells (such as neutrophils, lymphocytes, monocytes, and resident macrophages), proinflammatory cytokines, and activated platelets are important players in this scenario.
Current evidence suggests that historic risk factors for atherothrombosis can instigate production of reactive oxygen species such as hypochlorous acid or superoxide anion; such risk factors often accompany inflammation, promoting proinflammatory cytokines and elevating production of these pro-oxidants. Proinflammatory cytokines such as interleukin-1 or tumor necrosis factor-α as inflammatory mediators can act directly in healthy and disease stages. Cytokines also change the normal anticoagulant and pro-fibrinolytic properties of endothelium to an activated state that fosters thrombus formation and stalls fibrinolysis. The inflammatory mediator CD40 ligand can induce vascular cells and mononuclear phagocytes alike to produce tissue factor, a potent trigger for thrombus formation [10] and finally innate immune system activation.
Proinflammatory cytokines also elicit the acute phase response from the liver, through the intermediary interleukin-6, the “messenger cytokine.” The acute phase reactants include proteins involved in the causal pathway of atherothrombosis and vein thrombosis (e.g., fibrinogen or PAI-1) or soluble biomarkers such as C-reactive protein or serum amyloid [14].
Significance of Endogenous Fibrinolysis
The main components of endogenous fibrinolysis are tissue-type plasminogen activator, platelet activator inhibitor-1, plasmin–antiplasmin complex, D-dimer, TAFI, and lipoprotein (a) [19]. The mechanism of the fibrinolytic system at a first glance shows that thrombin converts the inactive proenzyme plasminogen to active plasmin. Plasmin degrades the cross-linked fibrin into soluble degradation products by the tissue-type plasminogen activator and the urokinase type plasminogen activators. It is tissue-type plasminogen activator that is mainly responsible for the dissolution of fibrin formed in the circulation. This fibrinolytic system can be inhibited either by antagonizing plasmin through α2-antiplasmin or by specific plasminogen activator inhibitors. There are three types of this inhibitor described so far; of these, physiologically, the most important one is the PAI-1 [19].
The thrombin activatable fibrinolysis inhibitor is another important inhibitor of the fibrinolytic system. This inhibitor forms a link between blood coagulation and fibrinolysis. Thrombin forms fibrin to stabilize the platelet thrombus and at the same time produces TAFI to protect the fibrin network. The inhibitor circulates as an inactive proenzyme and becomes activated by thrombin during blood clotting. Its active form inhibits fibrinolysis by cleaving off C-terminal lysine residues from partially degraded fibrin that stimulate the tissue plasminogen activator-mediated conversion of plasminogen to plasmin. Consequently, removal of these lysines leads to less plasmin formation and subsequently to protection of the fibrin clot from breakdown [19].
Although the plasmin-mediated fibrinolytic system is the major physiological mechanism of removing fibrin from the circulation, the plasmin-independent breakdown of fibrin by cellular components of blood also plays a significant role. An arterial and venous thrombus contains significant amounts of leukocytes (neutrophils and monocytes) covering the thrombus surface or incorporated into it, due to the interaction of activated platelets with leukocytes [10, 11]. Neutrophil membrane proteolytic enzymes (elastase, cathepsin G) can breakdown fibrin directly and also assist the dissolution of thrombus by plasmin. Furthermore, the physical properties of fibrin formed during hemostasis/thrombosis greatly affect the rate and effectiveness of fibrinolysis [18]. In the setting of VTE, the exact mechanism to explain endogenous fibrinolysis hypoactivity is unknown.
Linking Thrombosis with Inflammation
Vein thrombosis has been traditionally associated with red blood cell and fibrin-rich “red clot,” whereas arterial thrombi superimposed on atherosclerotic lesions with active inflammation are rich in platelets, giving the appearance of a “white clot.” Nevertheless, experimental and morphological studies suggest that inflammation and platelet activation also participate in venous thrombogenesis [7].
A relationship between inflammation and thrombosis has been identified in different clinical scenarios where the inflammatory process and coagulation abnormalities are clearly interlinked (septic shock and disseminated intravascular coagulation, as well as in the context of VTE). The molecular mechanisms that could explain this link are becoming apparent [31]. Inflammation is known to upregulate C-reactive protein expression and to modulate platelet function. Very importantly, it also affects the initiation phase and the fibrinolytic system, enhancing the thrombotic response to vascular injury in-vivo [32]. Evidence suggests that the complement system may serve as a link between inflammation and thrombosis and reveals specific interactions between complement proteins and platelets. The complement system was shown not only to induce platelet activation and aggregation but also to potentiate thrombin-induced platelet secretion and aggregation. At the same time, platelets can activate the complement system via P-selectin [4].
Interleukin-6 induces the expression of tissue factor, fibrinogen, factor VIII, and von Willebrand factor. Coronary and pulmonary vascular release of interleukin-6 and 8 has been observed in some clinical situations [7]. Such enhancements of cytokine and chemokine levels can lead to endothelial activation and endothelial cell damage, as well as increase platelet aggregation, sensitivity to thrombin, and recruitment and activation of leukocytes at the vascular wall. Ultimately, all of these precipitate localized formation of thrombin and fibrin [4, 7]. Moreover, an increased interleukin-6 level lowers the concentration of the natural inhibitors of hemostasis such as antithrombin, protein S [4] and thrombomodulin [22]. This mechanism could explain “unprovoked” or “idiopathic PE” acute index events in high-risk population.
Thrombin has a well-recognized role as a molecular effector at the interface of thrombosis and inflammation and the clotting cascade. Thrombosis can initiate proinflammatory responses central to the pathogenesis of atherosclerosis. Thrombin levels increase at sites of vascular injury and during acute coronary syndromes and has powerful proinflammatory effects on endothelial cells, smooth muscle cell, and platelets that promote the development of vascular disease. It also activates endothelial cells by cleaving and activating endothelial protease-activated receptor-1 and 2, which increase inflammatory gene transcription and stimulate expression of numerous leukocyte adhesion molecules including vascular cell adhesion molecule-1, intracellular adhesion molecule-1, and E-selectin through nuclear factor Kappa-ß and GATA-dependent transcription factor pathways [32].
The nuclear factor Kappa-ß is a pivotal transcription factor that may play a key role in plaque instability by promoting the expression of a cascade of procoagulant and proinflammatory genes in response to a variety of stimuli [33]. It has been identified in monocyte/macrophages, smooth muscle cells, and endothelial cells in human atherosclerotic vessels but not in healthy vessels. Additionally, in coronary plaques from patients with acute coronary syndromes, it exhibits increased expression of several of its genes-including proinflammatory cytokines, growth factors, chemokines, adhesion molecules, and TF [19].
Thrombin promotes endothelial secretion of pro-atherogenic chemokines such as monocyte chemotactic protein-1, platelet-derived growth factor, macrophage migration inhibitory factor, interleukin-6, and interleukin-8 and results in monocyte adhesion. Thus, production of thrombin-induced interleukin-6 by endothelial cells and smooth muscle cells generates a systemic prothrombotic response by increasing levels of circulating PAI-1 and fibrinogen. Thrombin activation induces cell retraction and reorganization of cadherins at endothelial junctions, thereby increasing endothelial permeability and promoting platelet deposition and leukocyte extravasation [31, 32].
CD40 ligand is a member of the tumor necrosis factor superfamily, primarily expressed on activated CD4+ T lymphocytes, but it is also found in a soluble form. CD40 ligand was originally described on T cells, but its expression has since been found on a wide variety of cells, including platelets, mast cells, macrophages, NK cells, B lymphocytes, vascular smooth muscle cells, and endothelial cells. The binding of soluble CD40 ligand to its CD40 receptor on the leukocyte can also induce tissue factor expression [4]. Vascular smooth muscle cells represent a link between inflammation and thrombosis, as the local thrombotic stimulation of smooth muscle cells in the arterial or vein wall can amplify the inflammatory response and increase fibrinogen and PAI-1 levels in the circulation [11, 12].
The behavior of platelets, polymorph nuclear granulocytes, and monocytes in normal human arterial and venous blood, as documented by flow cytometry and direct microscopic visualization, demonstrates the occurrence of platelet–leukocyte microaggregates in whole blood; the analysis of venous thrombi reveals tangled pale strands of aggregated platelets and fibrin within the mass of red blood cells. Induced venous thrombus in the presence of radio-labeled platelets shows early platelet accumulation at the “head” of the thrombus; then, the acquisition of platelets by the thrombi slows, and the clots become “red,” being predominantly composed of fibrin and erythrocytes. Another evidence of the participation of platelets in venous thrombosis comes from clinical studies in PE patients showing increased urinary excretion of thromboxane A2, a marker of platelet activation [7].
Inflammation as a Trigger
From a simple view, inflammation is one of the first responses of the immune system to infection. The symptoms of inflammation include redness and swelling, which are caused by increased blood flow into the tissue. Inflammation is driven by eicosanoids and cytokines, which are released by injured or infected cells. Common cytokines, which regulate inflammatory response, include interleukins (mainly interleukin-6) that are responsible for communication among white blood cells, chemokines that promote chemotaxis, and interferons that have antiviral effects.
Acute infections have been associated with a transient increase in the risk of myocardial infarction and stroke and recently with venous thrombosis as well, supporting the notion that systemic infections increase the risk of thromboembolic events [7]. Among the three precipitants proposed in Virchow’s triad, infection could affect venous stasis and increase blood coagulability. Furthermore, parallels with the arterial system suggest that damage to the vessel wall might not be limited to physical damage but could affect endothelial function as well. Inflammation is a key determinant of endothelial activation in arteries and veins (in health and disease states) and a link between infection and venous thrombosis [7, 10, 11].
Acute infections induce white-cell activation, dehydration, and bed rest as collateral thrombosis mechanisms; it is important to recognize that inflammatory and thrombotic pathways share common signaling pathways and that inflammatory responses promote activation of the clotting cascade and stimulate platelet activation [32]. Additionally, several studies have identified an increased risk of VTE in patients with non-infectious inflammatory disorders, such as inflammatory bowel disease and connective tissue disease. Considering the evidence, it is possible that [7] in high-risk populations [12, 14, 32] with atherothrombosis risk factors [3–5], an acute infection could trigger arterial and/or vein thrombosis through endothelial activation [10, 11, 31]. Vaccines to reduce or avoid respiratory infections and early detection of chronic infections (periodontitis, prostatitis, etc.) through office visits and C-reactive protein measurements could be used as new elements in the primary or secondary VTE prevention.
Inflammation directly or indirectly affects the venous endothelium inducing endothelial activity and inflammation-mediated vascular remodeling. In response to inflammatory events, endothelial cells produce inflammatory mediators (chemokines and cytokines), thereby recruiting inflammatory cells (macrophages, dendritic cells, mast cells, B cells, T cells, and T regulatory cells). With the coordination of inflammatory mediators, inflammatory cells might perpetuate the release of cytokines, chemokines, and growth factors [31]. Finally, these processes lead to vascular remodeling and vein thrombosis with or without a thromboembolic event. In any model of endothelial dysfunction the final pathway will be ischemia. In the model of endothelial dysfunction, venous thrombosis and PE complicated with acute pulmonary arterial hypertension (obstruction >30 %), the tissue damage occurs on the right ventricular endocardium, inducing ventricular dysfunction. In all models of pulmonary arterial hypertension, clinical symptoms, signs, and mortality are related to right ventricular dysfunction. Currently, in PE right ventricular dysfunction—with or without acute cellular damage—is a mortality risk marker, identifies submassive or high-risk PE and stratifies patients to fibrinolytic therapy [34–45].
Right Ventricular Myocarditis
Recently Begieneman and colleagues [46] in an elegant necropsy study showed the presence of inflammatory cells and myocytolysis related with intracavitary thrombi in the left and right ventricle of patients who died because of PE as a presumed new source of ventricular dysfunction. Previous evidences showed an increase in the level of CD68-positive macrophages in the right ventricle of PE patients and the influx of macrophages in the heart was attributed to ischemia [47, 48]. In these studies, inflammatory infiltrate and myocarditis were not discussed. Begieneman and colleagues [46] defined myocarditis as aggregation of inflammatory cells in the myocardium coinciding with areas of myocytolysis, conforming to the Dallas criteria for myocarditis. The presence of inflammatory cells in the endocardium of the heart was diagnosed as endocarditis. Compared with controls (no-PE and pulmonary arterial hypertension patients), extravascular foci of aggregates of lymphocytes, neutrophilic granulocytes, and macrophages were found dispersed in the right and left ventricle, coinciding with areas of myocytolysis, indicating myocarditis in acute PE patients.
In the same publication, a significant increase in the number of neutrophilic granulocytes, lymphocytes, and macrophages in the right ventricle (p = 0.012, p = 0.02, and p = 0.027, respectively) and a significant increase in the number of neutrophilic granulocytes and macrophages in the left ventricle (p = 0.034, p = 0.018, respectively) was observed. No significant difference was found between the number of neutrophilic granulocytes in the left and right ventricle (p = 0.352). However, the accumulation of lymphocytes and macrophages was significantly lower in the left ventricle than in the right ventricle in patients with PE (p < 0.001 and <0.001, respectively). To determine a putative relation between the age of the lung thromboemboli and the accumulation of inflammatory cells in the heart, patients were divided into two groups: thromboemboli <4 days old and thromboemboli >4 days old.
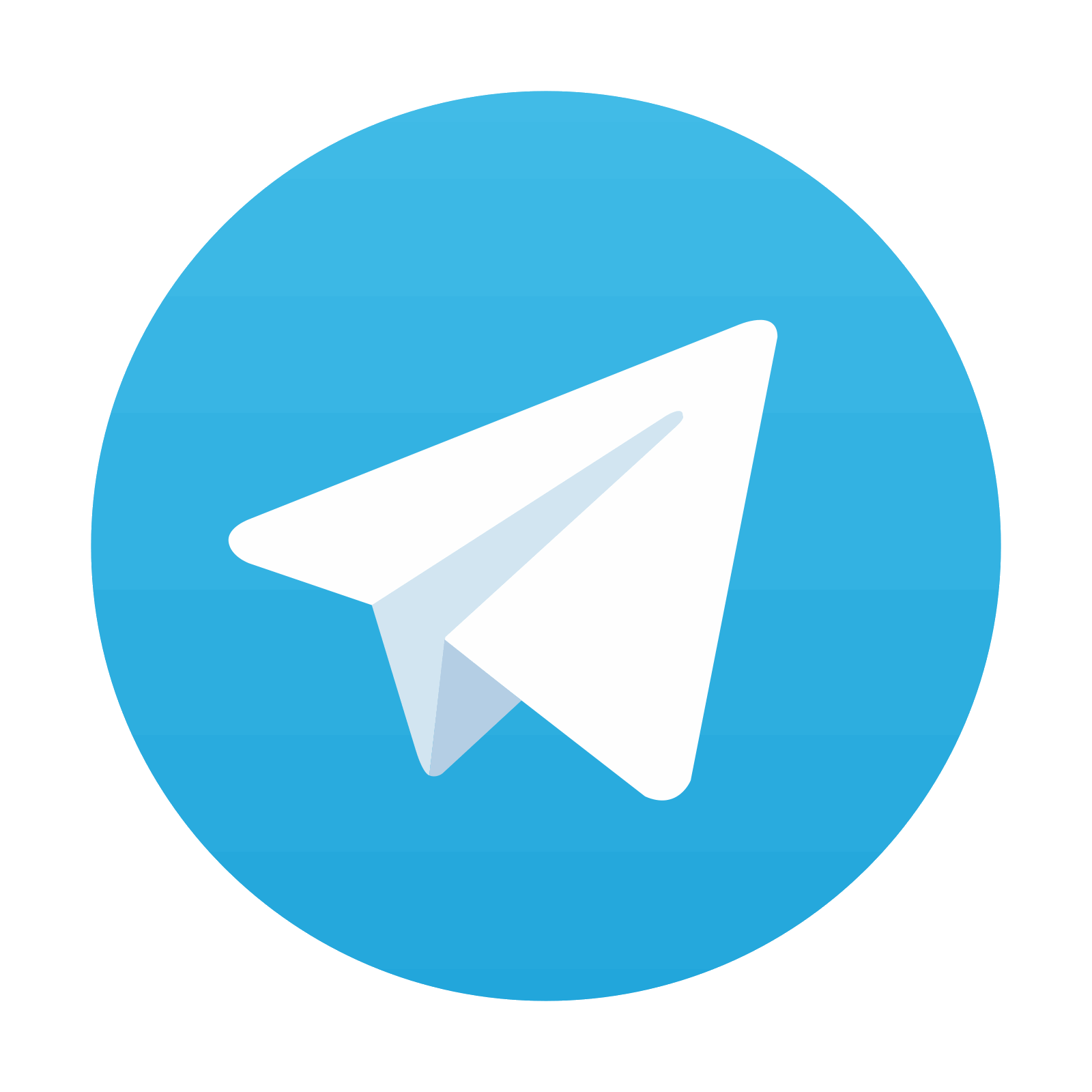
Stay updated, free articles. Join our Telegram channel
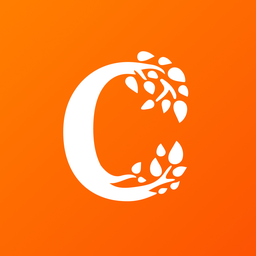
Full access? Get Clinical Tree
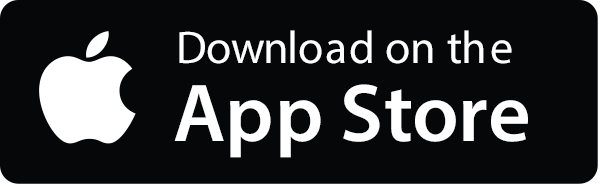
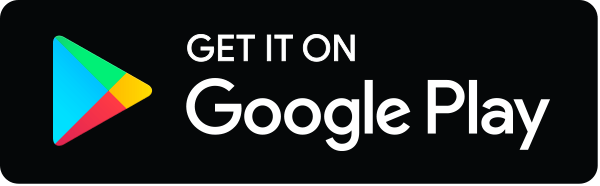