FIGURE 102.1 The effects of gravity on ventilation–perfusion ratio (VA/Q). In the upright subject, gravity affects VA, Q, and concomitantly, the VA/Q ratio. Gravitational forces pull on both the gases we breathe and pulmonary blood flow; as a result, VA and Q increase from a lung’s apex to its base. Because of blood’s greater mass, gravity’s effect upon Q predominates; it is estimated that Q at a lung’s base is six times as great as at its apex, compared to only 2½ times as much VA at the lung’s base versus its apex. The resultant VA/Q ratio is therefore greater at the lung apices than the bases.
Poliomyelitis, Guillain–Barré syndrome, and other neuromuscular disease states produce respiratory insufficiency because of mechanical and neural failure to control diaphragmatic-driven ventilation. In the absence of complications such as aspiration of gastric contents, pulmonary parenchymal function remains intact. By contrast, ARDS represents a failure of gas exchange that is related almost entirely to parenchymal involvement. Furthermore, neuromuscular and musculoskeletal function generally remains unimpaired during ARDS, although there are exceptions, such as flail chest associated with underlying pulmonary contusion after trauma. Even in this situation however, the musculoskeletal abnormality is of secondary importance compared with the underlying lung contusion.
Even the simplest ventilator provides a satisfactory means for sustaining VA when neuromuscular and musculoskeletal problems predominate. Yet, it would be very surprising if a simple ventilator performed equally well in the therapy of ARDS, in which an entirely different spectrum of pathophysiologic changes occurs. Thus, when a decrease in residual alveolar volume is present—whether caused by surfactant depletion, partial airway obstruction, interstitial and alveolar pulmonary edema, or a combination of these factors—a simple mechanical ventilator can have salutary effects only while it restores alveolar volume and improves oxygen exchange during the inhalation phase provided, of course, that ventilatory pressures do not approach or exceed pulmonary hydrostatic pressures. During exhalation, the beneficial effects of inflation quickly dissipate, especially if alveolar volume is allowed to return to its starting point.
In fact, opening alveoli during inhalation, and allowing them to close again during exhalation, may exacerbate the problem. It seems that when alveoli collapse, surfactant activity is lost; there are two putative theories to explain this: (a) surfactant molecules are forced into close proximity during collapse; ultimately they collide and clump together (9,10); and/or (b) surfactant is forced up, into the airways, during alveolar collapse (11)—once in the airways, surfactant is either damaged, or removed by ciliary action.
Positive End-Expiratory Pressure
Reduced levels of surfactant, such as occur during ARDS, lead to widespread atelectasis and hypoxemia; these conditions respond poorly to mechanical ventilation alone. The law of Laplace states that pressure inside a spherical structure is directly proportional to tension in that structure’s wall and inversely proportional to its radius. Normally, alveolar surface forces, at alveolar-capillary membranes, are essentially identical. Laplace’s law dictates that a loss of surfactant means a greater pressure is required to keep smaller alveoli open (Fig. 102.4). When this occurs, smaller alveoli empty into larger ones, eventually collapsing. A plot of the lung’s pressure–volume relationship, during ARDS, helps to better visualize this phenomenon (Fig. 102.5). Without adequate surfactant, significant portions of the lungs collapse at end-exhalation. During inhalation, as pressure is applied to the airways (x-axis), nothing initially happens. However, when the applied pressure reaches sufficient magnitude, in this instance 14 cm H2O, some of the collapsed alveoli start to open and gas begins entering the lungs. This “opening” pressure is commonly referred to as the lower inflection point, and provides the theoretical underpinnings for the use of positive end-expiratory pressure (PEEP). That is, an ARDS-related surfactant deficiency predisposes to alveolar collapse, unless counteracted by force. Clinically, the easiest way to accomplish this goal is by maintaining PEEP, preferably somewhat above the lower inflection point (12). Because the therapeutic objective is to prevent alveolar collapse—that is to say, keep the alveoli open—the approach is often referred to as the open lung approach (13,14).

FIGURE 102.2 Ventilation–perfusion (VA/Q) abnormalities. A: Absolute shunt—low VA/Q ratio. An intrapulmonary, or absolute, shunt occurs when blood continues to perfuse collapsed, or otherwise unventilated alveoli–blood literally shunts past, or bypasses the lung, without participating in gas exchange. B: Alveolar dead space—high VA/Q ratio. Dead space or wasted ventilation exists when alveoli receive ventilation but no blood flow.

FIGURE 102.3 Relative ventilation–perfusion (VA/Q) abnormalities. A: Relative shunt—low VA/Q ratio. A relative shunt occurs when an alveolus receives too much Q in relation to VA. B: Relative dead space—high VA/Q ratio. Relative dead space exists when an alveolus receives too much VA relative to its Q.
Combining mechanical ventilation and PEEP usually decreases shunt and improves oxygenation (15–18), often significantly; nevertheless, ARDS mortality rates have failed to improve. The reasons for this are complex, but theories for these failures are starting to emerge (19). It now appears that, to reduce ARDS-associated morbidity and mortality, we must avoid the risks associated with both low-volume (4–7) and high-volume (1–3) lung injury. To accomplish this, all tidal ventilation must occur between the lower and upper inflection points (19). This sounds easy; but bedside determination of inflection points is difficult; nevertheless, it’s worth the effort.
To date, a universally agreed upon ventilatory approach, or mode, for managing critically ill patients has failed to emerge. Considering the wide variety of conditions ameliorated by mechanical ventilation, and the extreme range of severity between patients with the same problem, a single, always best, approach may not exist. Clinicians, therefore, must understand and recognize the potential and limitations of their favored approaches.
VENTILATOR CLASSIFICATION
Positive versus Negative Pressure
Today, virtually all ventilators function by providing some variant of positive pressure. Yet, during the polio epidemic, “iron lungs” or negative pressure ventilators were in common use. Negative pressure devices require that the patient’s body be tightly enclosed within a tube or box, while the head remained outside. Once the patient is sealed inside, a pump or bellows evacuates gas, from inside the box; this creates a negative pressure around the patient’s thorax, making atmospheric pressure positive in relation to alveolar pressure. As a result, gas flows from the mouth to alveoli, trying to equalize the pressure difference. Because this process is nearly identical to normal breathing, negative pressure ventilators tend to provide better VA/Q ratios (20) and produce less interference with cardiac output (21) than positive pressure counterparts. Nevertheless, these devices quickly lost favor for a number of compelling reasons: (a) iron lungs are very large and difficult to move; (b) maintaining an air-tight seal around the patient’s neck, without irritation, is nearly impossible; (c) personnel responsible for providing patient monitoring and routine care could not easily access important areas of their patient’s body.

FIGURE 102.4 Laplace’s law and its effect on alveoli. Laplace’s law states pressure (P), inside a sphere, is directly proportional the tension in the walls (T) and inversely proportional to the sphere’s radius. A: Without surfactant. Wall or surface tension in both large and small alveoli is about the same. As a result, a greater pressure develops in the smaller alveolus, which then proceeds to empty into adjacent, larger alveoli. B: With surfactant. The surface tension reducing properties of surfactant increase as individual surfactant molecules get closer together. This property counteracts Laplace’s law, and reduces the tendency for small alveoli to empty into nearby larger alveoli.

FIGURE 102.5 Inflation and deflation characteristics in the surfactant-deficient lung. Surfactant deficient alveoli generally remain open throughout exhalation (open circles); at end-exhalation, unstable alveoli empty into adjacent, larger alveoli and collapse. This phenomenon reduces functional residual capacity (FRC), often significantly. Furthermore, once collapsed, alveoli tend to stay collapsed until a relatively high pressure is applied. In this idealized example, airway pressure is steadily increased to inflate the lungs (solid circles). Note airway pressure reaches 14 cm H2O, before any measurable volume enters the lungs; at this point (lower inflection point), collapsed alveoli begin to open, the lungs begin to accept volume, and the pressure–volume curve changes slope upward. Alveoli continue to open, those already open expand, and airway pressure continues to rise until the average patent alveoli begin to approach their maximum volume. At this point, the pressure–volume curve flattens—in other words, from this point on, larger changes in pressure will be required to produce a complimentary change in volume. This slope change is referred as the upper inflection point. Although the pressure–volume curve may be difficult to measure at the bedside, avoiding ventilator-induced lung injury requires that all mechanical ventilation occur between the upper and lower inflection points; that is, patient airway pressure should not be allowed above the upper inflection point, or below the lower inflection point.
Controlled versus Assisted Breaths
Although modern ICU ventilators offer many different operational modes, from the patient’s standpoint, only two breath types remain: controlled (mechanical or mandated) and assisted spontaneous. Controlled breaths, used during controlled mechanical ventilation (CMV), are completely defined by the attending clinician. Controlled breaths are always delivered on schedule and without regard for the patient. CMV strategies should replace 100% of a patient’s work of breathing (WOB). However, patients allowed to breathe spontaneously during CMV frequently end up out of phase—that is to say, attempting to breathe when the ventilator is not in the inspiratory phase—with the ventilator. Also known as dyssynchrony, out of phase breathing during CMV produces very high patient WOB (22). Assisted-spontaneous breathing strategies involve a work-sharing approach between the patient and ventilator (23). Theoretically speaking, a work-sharing approach makes perfect sense; ideally, the ventilator functions to “unload” WOB the patient cannot tolerate. Critically ill patients face an above normal workload, primarily from their pulmonary disease process and, secondarily from their artificial breathing apparatus, including the endotracheal tube (ETT), breathing circuits, humidifiers, and the ventilator (24,25). Unfortunately, there is a fatal flaw in the ventilator–patient work sharing concept: until recently (26) we have not been able to find a reliable, readily available, easy-to-perform, and noninvasive methodology for determining just how much WOB our patients can actually tolerate (27–29), and this determination is absolutely crucial. If the ventilator off-loads too much work, the patient’s respiratory muscles are predisposed to atrophy. If the ventilator provides insufficient support, fatigue is likely. Either scenario can add unnecessary days, or even weeks, to the period time patients require ventilatory support. Fatigued or weak patients make poor candidates for weaning and attempts at liberation from the ventilator; moreover, the risk for developing ventilator-associated pneumonia (VAP) correlates directly to the time spent receiving ventilatory support (30). Research suggests that the diaphragm, which evolved to contract without interruption from birth until death, begins to loose contractility shortly after initiating CMV (31); the loss of contractility is time-dependent and continues to worsen as mechanical ventilation is prolonged (31).
Ventilator Breaths: Defining Characteristics
The idea of trying to classify each ventilator type—to better understand how specific ventilators interacts with and affect physiology—remains as common today as ever. Yet, today’s ventilators include so many modes and options that they are near impossible to classify. For a time, some tried to classify ventilator modes (32), but even this strategy is no longer reliable because many ventilators now incorporate dual-mode capabilities—the ability to switch modes within an individual ventilator breath.
Instead of trying to classify ventilators, modes, or even submodes, it may be easier to develop and use a standardized set of terms, and describe the breath types in use. This is possible because, regardless of ventilator or breath type, all ventilator breaths are delivered in four distinct phases or variables (Fig. 102.6).
Phase or Control Variables
Each ventilator breath must begin, for some reason and at some specific moment in time. The physical change that initiates a breath is known as the trigger variable (labeled A in Fig. 102.6). Once a breath is triggered “on,” the ventilator must somehow, precisely manage how gas is forced into the patient’s lungs; the physical characteristic managed during lung inflation is the control variable (labeled B in Fig. 102.6). After delivering the prescribed volume or pressure, inflation must end. This physical change responsible for ending inflation is called the cycle variable or cycling (labeled C in Fig. 102.6). Immediately following inhalation is exhalation, or the exhalation variable (labeled D in Fig. 102.6). Unlike the previous three variables, use of an exhalation variable is optional.
In early attempts at ventilator classification, author(s) often used the term cycling for both breath initiation and termination. Do not confuse the breath initiation, triggering, with breath termination, cycling; the ambiguous use of the term “cycling” continues to produce confusion.
All ventilators systematically, change the pressure, flow, and volume with respect to time. It follows that, when characterizing any of the four phase variables, pressure, flow, volume, or time are the only possible physical characteristics a ventilator could change or control, during any phase of respiratory cycle (Table 102.1). It is also important to note that while some of these characteristics correlate and may change together, in direction and magnitude, they do not always do so. The practical side of this translates into an important tenet: ventilators can only control one characteristic at a time. This does not however, preclude using multiple characteristics, provided they are used in a logical sequence, and one at a time.

FIGURE 102.6 Pressure, flow, and volume curves for a mechanical ventilator breath. A: The trigger variable. The trigger variable is the physical characteristic used, by the ventilator, to initiate a mechanical inflation of the lungs. In this case, pressure falls before the breath starts; the represented ventilator breath is likely pressure-triggered. B: Control variable. For patient safety, the ventilator must precisely control an important aspect involved with inflating the patient’s lungs. For this breath, flow is held constant; the ventilator is described as flow-controlled. C: Cycle variable. Each mechanical breath must end such that the lungs are properly filled and then allow the patient to exhale. The physical characteristic that determines appropriate lung filling, is the cycle variable. This example shows the breath ending after the control variable (flow) has continued for a specific interval of time; this ventilator is time-cycled. D: Expiratory variable. Modern ventilators either control pressure during exhalation or they do not. In this example, pressure returns to ambient (0 cm H2O), so this ventilator has no operative expiratory variable.
TABLE 102.1 Ventilator Breath Classification | ||
![]() |
Trigger Variable
Ventilators are triggered “on” by time, pressure, flow, or volume. Today’s ventilators however, often depend upon multiple trigger variables, used sequentially. For example, with a trigger sensitivity set for 2 (actually, –2 cm H2O), breath rate set to 10 breaths per minute (bpm), and in the CMV mode, this ventilator has, at least, two trigger variables. First, breaths may be pressure-triggered when the ventilator is in the expiratory phase and baseline airway pressure is reduced by 2 cm H2O, or more. If, however, the patient makes no attempt to breathe; the CMV rate timer counts reaches 0 and a time-triggered breath results—exactly 6 seconds(s) after the last ventilator breath. This mode is known as assist-control (A/C), because patients can assist as often as they like, but if they stop breathing (assisting), the ventilator reverts to the predefined CMV rate. Another way ventilators employ multiple trigger variables is by utilizing two sensors, for instance pressure and flow; triggering occurs in response to the variable’s threshold (pressure or flow), breached first.
Control Variable
Once a breath is triggered “on,” patient safety cannot be assured unless the delivery of gas into the lungs is precisely controlled. Of the four potential physical changes (Table 102.1) only pressure and flow are used to any extent. Flow is emphasized to underscore an engineering issue: volume-controlled ventilators are actually flow-controlled. There are several reasons for this. First, the integral of flow, with respect to time, is volume. It follows that by precisely controlling inspiratory flow (VI), for a preset inspiratory time (TI), produces an exact tidal volume (VT), based on the following equation:
Algebraically speaking, the operator can preset only two of these variables, in this case VI and TI; the third variable (VT) cannot be preset; it is a consequence of VI and TI. Given a choice however, most clinicians prefer to preset the VI and VT; in this situation, TI becomes the consequential or resultant variable. In an effort to obviate this preference, most of today’s ventilators use an algebraic variant of equation 1:
This design allows operators to preset VT and VI. Furthermore, because the operator actually predefines VT —referring to the ventilator as a volume-controller, or as volume-controlled ventilation (VCV)—this is perfectly acceptable; this nomenclature will be used henceforth.
Volume-controlled strategies differ markedly from pressure-controlled ventilation (PCV). When we opt for VCV, our priorities are clear: we wish to prescribe (preset) VT, VI, and flow pattern. If we wish these parameters reliably delivered, then airway pressure (Paw) must not be restricted. When Paw is allowed to vary, VT, VI, and flow pattern are delivered, regardless of the patient’s pulmonary mechanics (Fig. 102.7). High peak inflation pressures (PIP) are a concern; so, ventilators allow clinicians to preset a maximum, safe level of Paw; this setting, referred to as a high pressure limit, which functions as a cycle variable—that is, ending inspiration (or diverting gas flow), the moment Paw violates the established threshold. Keep in mind though, cycling via high pressure limit truncates breath delivery, negates volume-control, and reduces VT.
Pressure-controlled strategies allow us to preset a desired Paw and TI; conversely, VT, VI, and flow waveform cannot be predetermined. Pressure-controlled breaths always generate an exponentially decelerating flow pattern; the individual’s CRS and Raw determine the magnitude of VI and VT (Fig. 102.8) (33,34). Inasmuch as PCV does not control VT, and preset pressure never varies, clinicians must insure PCV is carefully monitored; always carefully set low/high VT alarms (if available), as well as low/high minute ventilation alarms.
Cycle Variable
All four physical changes are commonly used for cycling ventilator breaths. Pressure-cycling is common during intermittent positive pressure breathing (IPPB); flow-cycling predominates during pressure support ventilation (PSV) and, either time or volume is common during VCV. Without question, time is the most commonly used cycle variable, particularly if one remembers that with today’s VCVs, cycling occurs when TI lapses, not in response to delivered volume.
Expiratory Variable
The expiratory phase is the least varied of the four; attempts at manipulating the expiratory phase variable have met with little success. Varying flow resistance (retard), negative end-expiratory pressure (NEEP) and PEEP have all been thoroughly tried and discarded. Compelling evidence however, substantiates using continuous positive airway pressure (CPAP) and PEEP to restore or increase functional residual capacity (FRC) (35–37), reduce shunt and improve oxygenation (15–18), and reduce WOB (38).

FIGURE 102.7 Response of volume-controlled ventilation (VCV) to a sudden change in respiratory system compliance (CRS). A: Compliance = 50 mL/cm H2O. An airway pressure, flow, and tidal volume curve for a patient with this CRS and receiving VCV. B: Compliance = 10 mL/cm H2O. An airway pressure, flow, and tidal volume curve, for the same patient as shown in panel A except, the patient’s CRS is acutely reduced. Note volume and flow are essentially unaffected, but, airway pressure is dramatically elevated as the ventilator required far more pressure to provide the same flow and volume into the much stiffer lungs.

FIGURE 102.8 Response of pressure-controlled ventilation (PCV) to a sudden change in respiratory system compliance (CRS). A: Compliance = 50 mL/cm H2O. An airway pressure, flow, and tidal volume curve for a patient with this CRS and receiving PCV. B: Compliance = 10 mL/cm H2O. An airway pressure, flow, and tidal volume curve, for the same patient as shown in panel A except, the patient’s CRS is acutely reduced. Note pressure is essentially unaffected, but, flow and volume are dramatically reduced as the far stiffer lungs accept much less flow and volume at the same airway pressure.
Classifying Breaths
The four phase variables (trigger, control, cycle, expiratory) provide us with an easy-to-use and understand method for classifying ventilator breaths. It makes sense classifying by breath behavior because today’s ventilators offer so many breath types. For instance, the breath depicted in Figure 102.6 is pressure-triggered, volume-controlled, and time- or volume-cycled; there is no exhalation phase variable. In Figures 102.7 and 102.8, the breaths are time-triggered, volume-controlled and time- or volume-cycled and, time-triggered, pressure-controlled, and time-cycled, respectively. Some ventilator modes like intermittent mandatory ventilation (IMV) or synchronized IMV (SIMV) allow two different breath types. Mandated breaths might be time-triggered, volume-controlled and time-cycled; while in-between scheduled breaths, spontaneous breaths might be pressure-triggered, pressure-controlled and pressure-cycled.
VENTILATOR DESIGN
Modern ICU ventilators are expensive and seemingly complex; yet, while the electronics may be complicated, basic ventilator component configuration is simple and has changed very little over the past 20 years. In simplest form, a mechanical ventilator requires only a few essential components (Fig. 102.9).
Power Sources
Pneumatics
Ventilators must have power. Most patients require, at least, some oxygen; this makes the energy stored within compressed oxygen a reliable and convenient power source. Gas-powered ventilators are called pneumatic. The powering gas source can be oxygen or compressed air, as long as the gas source is free of contaminants, debris, and is dry. Hospital oxygen supplies virtually never pose contamination or water concerns; whether in bulk form or in cylinders, oxygen is certified clean and pure (99.99%). Compressed air sources are however, a completely different matter. Compressors aspirate air from the environment; if aspirated air is contaminated, so too will be the compressed air. There have been instances of hospitals locating compressor intakes too close to parking lots and, on occasion, compressing exhaust gases along with air. Also, environmental air contains water vapor, some of which condenses and becomes liquid during the compression process; any and all water must be removed or it can cause serious damage to ventilators and other pneumatic equipment. Finally, most compressors use rapidly moving pistons or rotors to compress the air; operating at such high speed requires lubrication. Compressed air, for human consumption, should never involve using an oil-lubricated device. Small oil particles are compressed along with the air and can cause serious lung injury, if inhaled.
Despite potential drawbacks associated with using compressed air sources, pneumatic ventilators offer several advantages, particularly when used for transport. For instance, a pneumatic ventilator is always ready to go; they never require time-consuming recharging as battery-powered units do. Moreover, pneumatic ventilators use no expensive batteries, power supplies, or electric cables that can fail or must be periodically replaced. Furthermore, batteries often contain extremely toxic components—lead, cadmium, lithium, and so forth—and must be properly disposed or recycled, often at hospital expense. Pneumatic ventilators are also exceptionally robust; many pneumatic components will operate through many millions of actuations without failure. They are also reasonably priced and easy to maintain.

FIGURE 102.9 Schema of a “basic” ventilator. This schematic includes all of the major components necessary for ventilator operation. The logic component provides timing signals responsible for the inspiratory and expiratory phases. Ventilator logic must also synchronize the onset of each breath by the closing of the exhalation valve. Ventilator logic may be provided by fluidics, analog electronics, digital electronics (microprocessors), or pneumatics. All ventilators, regardless of simplicity, are either electrically powered with or without battery back-up, or pneumatically powered. None so far are powered by both.
Electric Power
Electricity is cheap, reliable, and, in most countries, virtually ubiquitous. As a result, electricity powers most ventilators. Electrically powered units utilize alternating current (AC), AC converted to direct current (DC), battery, or some combination (see Fig. 102.9). Unfortunately, ventilators are either pneumatically or electrically powered, never both. As a result, if power outages are likely, clinicians must consider their alternatives carefully; ventilators with battery back-up are great, but will only operate for, at best, a few hours. Few, if any of us have considered how ventilator-dependent patients would be ventilated in the event of an extended loss of electricity. This point is not simply a theoretical one, as just such a scenario occurred following hurricane Katrina (39).
Conventional Ventilator Logic
All ventilators require some sort of logic to coordinate the timing of inhalation (I) and exhalation (E), as well as actuating the flow/volume delivery mechanism and the exhalation valve (see Fig 102.9).
Traditionally, ventilator logic involved pneumatics, standard electronics, fluidics, or some combination of these. To initiate and maintain inhalation, logic signals simultaneously activate both the flow/volume delivery system and the exhalation valve. At the same time, ventilator logic is responsible for timing or controlling inhalation and, for monitoring breath delivery; the ventilator’s logic must be prepared to cycle the breath “off” if the high Paw limit is breached, or when cycling criteria is met.
Microprocessor-Controlled Logic
The first microprocessor-controlled ventilator was introduced in the early 1980s. Today, microprocessor-controlled logic dominates virtually every category of mechanical ventilation. Given that a microprocessor, or central processing unit (CPU), has virtually no influence on ventilator performance per se; it’s not unreasonable to wonder why microprocessor ventilators are so popular. The answer is, in a nutshell, that they are far more flexible and vastly safer than any other type of ventilator. Some of the many advantages offered by today’s CPU-controlled ventilators are listed in Table 102.2.
Relational Logic
An advantage offered only by a CPU is an ability to answer relational questions. A CPU can easily evaluate the “truth” of simple relational expressions such as: is x < y?, x = y?, or is x > y? A relational question can either be true or false. For instance, x might be the patient’s Paw and y the operator-selected high pressure limit. During each breath, the CPU could be instructed to evaluate, every few milliseconds, the relational expression is x > y? If the answer is false, then the ventilator breath continues; if the answer is true, then the breath would cycled “off” and the over-pressure alarms sounded and/or illuminated. Modern CPUs evaluate simple expressions with blinding speed. In fact, microprocessor-controlled ventilators evaluate dozens of relational expressions, in a specific sequence, continuously, until each mechanical breath is safely delivered.
TABLE 102.2 Advantages of Microprocessor-Controlled Ventilators |
![]() |
Logical Expressions
Microprocessors can also evaluate logical expressions or operate on the results of a relational question; logical operations follow the rules of Boolean algebra. For example, NOT true is false and NOT false is true. The AND function operates on two relational questions and requires that they both be true for the result to be true. That is, true AND true is true, but true AND false is false. The OR function also operates on two relational questions but only requires one of the questions to be true for the result to be true; true OR false is true and false OR false is false.
As an example, a CPU might evaluate the following two questions: Is exhaled VT less than inhaled VT, divided by 2? AND is the operator setting for VT unchanged? If answered true AND true, then the ventilator might be instructed to warn clinicians of low exhaled VT; from there the patient’s breathing circuit and ET cuff could then be quickly checked for leaks. With these simple building blocks, powerful algorithms can be devised that monitor all aspects of ventilator operations and make today’s mechanical ventilators safer than ever before.
Computer Memory
A CPU, no matter how powerful or fast, cannot function without memory. How could a CPU answer the relational question: “Is x (Paw) greater than y (airway pressure limit),” if it couldn’t remember the value of x or y? Additionally, how would a CPU know when, or how often, to answer relational questions?
In our example, the value for x (Paw) varies continuously as a function of time, while the value for y (airway pressure limit) may remain constant; on the other hand, y will most likely vary from one patient to the next. Somehow, the CPU must be able to update the values for x and y as often as they change. This requires, easily erasable memory, known as random access memory (RAM). There is a caveat, however: easily erased means volatile, and volatile means easily lost. For instance, valuable data might be lost the instant power is lost. As a result, ventilator CPUs cannot operate safely without battery backup to maintain critical data stored in RAM; without a patient’s exact data safely stored, the ventilator could malfunction, even if power was lost for an instant. Data stored in RAM is bidirectional; this means the CPU can store (write) information into RAM and read it later. Memory is limited, so the CPU must use its memory over and over again. Suppose the area used for storing a patient’s pressure limit (y) is already “occupied” and the operator changes the pressure limit; the CPU simply “writes over”—thereby erasing—the pre-existing pressure limit value.
Instructions for how to use data stored in RAM, and the sequence and timing of all functions carried out by the CPU, reside is a different type of memory known as read only memory (ROM). This form of memory is nonvolatile and not easily altered. It is this memory that queues the CPU as to how often and when to evaluate the relational and logical operations. In fact, the entire sequence, or code, responsible for every conceivable ventilator function is stored in ROM. The use of ROM comes with a caveat too: ROM is nonvolatile; but it is not impervious. Even the slightest change in a critical instruction could harm a patient. As a result, ventilator CPUs must have powerful “watchdog” systems that constantly evaluate every aspect of their behavior. Watchdogs always err on the side of safety—should they detect anything out of the ordinary they immediately terminate CPU operation, protect the patient, and alert the operator of the malfunction, often referred to as a vent-inop. Once a “bizarre” or unusual behavior has been detected, manufacturers insure that CPU integrity be verified before the ventilator will function again. Unfortunately, too often, vent-inop conditions require technical assistance from a biomedical engineer or factory representative. To obviate such problems, engineers have tried two, or even three, CPUs, which are programmed to constantly evaluate each other. This strategy eliminates the need for watchdogs; but, in the case of only two CPUs, does not eliminate the problem: when one CPU detects a “problem,” who decides which CPU is still functioning properly? With a three CPU design, there is always a “referee”; the aberrant CPU, once detected can be shut off leaving two CPUs to continue safely operating the ventilator until it can be safely replaced.
Ventilator Control Systems
Open-Loop Control
Open-loop ventilator designs (Fig. 102.10) are economical and straightforward, but functionally limited. Ventilators employing open-loops offer VCV or PCV; they seldom provide both. Open-loop systems are also not fault-tolerant. For instance, suppose over time and with prolonged usage, a ventilator’s flow valve gradually drifts out of calibration. Now, the signal designed to produce 0.75 L/s, yields only 0.60 L/s and VT is preset to 0.75 L. This patient will receive a VT no greater than 0.6 L, and the ventilator, even if CPU controlled, would have no way of detecting this problem.
Closed-Loop Control
Closed-loop or feedback designs (Fig 102.11) are far more complex and expensive than open-loop designs. In return, they deliver exceptional accuracy and automatically correct for many common failures and variances. Using the same preset VT of 0.75 L and VI of 0.75 L/s, a closed-loop ventilator delivers the requested VT even if the flow-valve is no longer calibrated—thereby protecting the patient. Given the example above, ventilator logic opens the flow valve, expecting 0.75 L/s; yet, a flow sensor, located just downstream from the flow valve, measures the actual flow (0.6 L/s) and sends an electric signal, proportional to measured flow, to the comparator (see Fig. 102.11). The comparator functions to analyze (electrically) the difference between the measured flow and actuating signals; if the signals are identical nothing happens, if the signals vary, the comparator provides an output signal, proportional in magnitude to the difference. The comparator’s output adds to, or removes from, the existing signal actuating the valve–in this case, the combined signals open the valve to produce a higher flow. Comparators function nearly instantaneously; so, the flow valve’s output can be corrected repeatedly, as often as the valve’s response time and the programmed TI allow. A response time of 10 ms, allows a 100 corrections in a TI of one second, if necessary.
Closed-loop feedback also corrects the ventilator outputs when affected by changing pulmonary impedance, different breathing circuits, and high resistance humidifiers. Closed-loop designs that incorporate flow and pressure sensors do not require separate valves for VCV and PCV. In this instance, a flow valve is either opened and a prescribed VT delivered, or the valve is opened to provide an initial high flow and a closed-loop pressure algorithm maintains any desired target pressure by manipulating the valve’s output flow based upon the target pressure.
Closed-loop designs require accurate, onboard flow and pressure sensors as well as sophisticated control algorithms, these are relatively costly and can be damaged by rough handling. For these reasons, transport ventilators often incorporate open-loop designs.

FIGURE 102.10 Schema of open-loop ventilator control. Virtually all positive-pressure ventilators control flow (shown) or pressure during each mechanical breath. The simplest control system involves a properly synchronized signal, from the logic element, that produces the output (flow); the ventilator does not verify accuracy so the operator must do so.

FIGURE 102.11 Schema of closed-loop ventilator control. Ventilator reliability and accuracy is vastly improved by measuring the actual output (flow), comparing the measured to desired, and correcting the actuating signal by the difference. At the onset of each breath, a signal from the ventilator’s logic actuates (opens) the output valve. The resultant output (flow) is measured immediately by a flow sensor positioned downstream. The flow sensor converts measured gas flow into an analog electric signal, which is routed to one side of an electronic comparator. The actuating signal (actual) from the logic element is fed into the other side of the comparator, where it is compared to the measured signal. If the two signals differ, the comparator adds (or subtracts) an amount of electricity, proportional to the signal difference, to (or from) the actuating signal. The entire loop requires only about 10 milliseconds to complete; that means the actual signal could theoretically be “corrected” as many as 100 times in a typical mechanical breath lasting just 1 second. Normally however, it only requires a few iterations before the measured and desired signals are identical.

FIGURE 102.12 Schema of a microprocessor-controlled, closed-loop control system. First-generation microprocessor-controlled ventilators combined digital (D) signals converted to analog (A), with an analog closed-loop system. This approach was necessary because digital control of closed-loop feedback added several time-consuming steps: corrected signals had to be converted D to A before they could operate ventilator valves; the measured signal had to be converted A to D before the microprocessor could compare it to desired and determine an appropriate correction. Unfortunately, microprocessors available at the time were simply not fast enough to adequately monitor lung inflation and provide corrected closed-loop signals. Today’s microprocessors easily perform billions of operation/second, and most second- or third-generation microprocessor-controlled ventilators provide closed-loop control using only digital signal processing.
Microprocessors and Closed-Loop Control
The first CPU-powered ventilators were too slow to perform all of the tasks involved in operating the ventilator and provide the corrected signals required for closed-loop control. To maintain accuracy and speed, the first generation of CPU-powered ventilators combined digital logic with analog, closed-loop control systems. In contrast, today’s CPUs perform billions of operations/second, leaving adequate time for the CPU to provide “corrected” signals necessary for closed-loop control (Fig. 102.12).
Microprocessors operate using digital (D) signals, but the majority of our real-world hardware (valves, sensors, transducers) require analog (A) signals. It follows that for a CPU to actuate a valve, a digital signal, from the CPU, must first be converted to analog; this takes place in a D to A signal processing chip (see Fig. 102.12). Analog information, such as a measured flow signals, must be similarly converted, A to D, before the CPU can use them. During closed-loop control, once a measured signal is converted, A to D, and reaches the CPU, it is compared to the current actuating signal; based upon the difference the CPU computes a new actuating signal; its digital representation is sent to the D to A; from there it proceeds to replace the existing signal. As each of these transformations take time, it is easy to see why early CPUs did not have sufficient speed to provide closed-loop corrections. The most recent CPUs are so fast, digital control rivals that of analog comparators for controlling closed-loop systems.
Future of Closed-Loop Control
In theory, by using a closed-loop algorithm, most modern ventilators could easily control any patient or ventilator parameter, provided there is a safe, reliable signal representing that parameter. That is, a ventilator could raise or lower the breathing rate, or VT, in order to maintain a target CO2 level; or, the ventilator might raise or lower the fractional inspired oxygen (FiO2), or CPAP level, to achieve a specified, target oxygen level. These possibilities still remain on the horizon, as the FDA ponders whether or not closed-loop control of such critical patient parameters will ultimately be as safe as the current generation of ventilators. Although some argue that the FDA is being overly cautious, it’s easy to imagine the potential for harm should a future ventilator fail to properly manage a life-sustaining parameter such as CO2 or O2. Nevertheless, the topic of whether future ventilators should be managed by computers or by experienced clinicians has become very popular at many of today’s respiratory therapy meetings. There are strong emotions and arguments from both sides: yet, it seems almost inevitable that computers will ultimately be allowed to control most or all of a patient’s vital parameters. Primarily, this is because educating, training, and providing the necessary experience to provide for sufficient numbers of respiratory therapists is an expensive and daunting task.
CONVENTIONAL MECHANICAL VENTILATORY TECHNIQUES
Compliance and Resistance—The End-Inspiratory Plateau
Operational Principles
The terms postinflation hold, end-inspiratory pause, and end-inspiratory plateau (EIP) all refer to the same ventilator routine; instead of allowing the patient to exhale the instant VT delivery is complete, the ventilator stops gas flow but does not allow the patient to exhale until a pre-specified period time, the EIP, elapses (Fig. 102.13). The EIP is considered part of TI because the VT volume remains in the lungs and the patient does not exhale until the EIP is complete; ventilators often allow EIP to persist as long as 2 seconds. Although this may not seem excessive, when combined with the existing TI, EIPs are often long enough to adversely impact hemodynamics and are poorly tolerated by spontaneously breathing patients.
Clinical Applications
An EIP has been advocated as a method to improve the distribution of inhaled gases, thereby decreasing VD/VT and PaCO2 (40). Theoretically, this makes sense; if inhalation was long enough, gas redistribution into slow-filling spaces would improve overall distribution (41). Gas redistribution during EIP, is thought to result secondary to collateral ventilation and Pendelluft flow.
Collateral ventilation occurs when gas enters alveoli from adjacent alveoli through channels in the alveolar walls (Pores of Kohn) or through cross-communications between bronchioles (Lambert canals). Pendelluft flow occurs when, during EIP, volume from fast-filling spaces redistributes into slow-filling spaces. Such gas flow is caused by regional pressure gradients that arise as a consequence of maldistribution secondary to positive pressure inflation.

FIGURE 102.13 Compliance and resistance determination in the ventilator patient. Respiratory system compliance (CRS) and airway resistance (Raw) determination, by end-inspiratory plateau (EIP), requires volume-controlled mode, square flow pattern, inspiratory flow rate (VI) of exactly 1 Liter (L)/second (s) (or, 60 L/min), and an EIP of 0.25 second or more. In this example, the peak inflation pressure (PIP) reaches 25 cm H2O, the instant flow ceases. After the preset tidal volume (VT) is delivered, the EIP begins, that is, gas flow from the ventilator ceases and the patient is not permitted to exhale. During the EIP, pressure equilibrates between the lungs and breathing circuit and elastic recoil pressure (ERP) of the lungs can be measured at the airway opening. The greater the difference between a patient’s PIP and ERP, the greater the Raw. Compliance is computed by dividing VT (0.65 L) by measured ERP minus the baseline pressure (0 cm H2O), and is given in units of L/cm H2O or mL/cm H2O. Resistance is computed as PIP minus ERP divided by VI (must be 1 L/s) and is stated in units of cm H2O/L/s. In this example, CRS is 0.038 L/cm H2O and Raw is 8 cm H2O/L/s.
The EIP is seldom used to improve distribution, but rather to determine CRS and Raw. During the plateau time, as gas flow ceases, the flow resistive component of PIP disappears. The remaining pressure, the plateau pressure, also reflects the static elastic recoil pressure (ERP) of the lungs. Exhaled VT, PIP, and ERP are used in determining the patient’s CRS and Raw (Table 102.3). These measurements are often performed routinely to assess the patient’s progress or gauge the response to bronchodilators.
Inspiratory Flow Waveforms
Before the advent of microprocessor-powered ventilators, different ventilator brands delivered gas flow using a wide variety of methodologies: pistons, injectors, bellows, solenoids, and so forth. Each flow generating technique produced a different inspiratory flow patterns: square or constant (Fig. 102.14A), sinusoidal, decelerating (Fig. 102.14B), or accelerating. Clinicians immediately began to wonder which waveform was best, or, could matching specific waveforms with specific pulmonary conditions make a difference? To this day, these questions remain essentially unresolved. Some tried various waveforms and found little or no difference in the distribution of ventilation (42). Other studies, modeling multiple lung compartments with different Raw, showed improved distribution with the decelerating waveform compared to others (43,44). Clinical reports confirmed the utility of a decelerating pattern (45–47). In one investigation, VT, TI, I:E ratio, and ventilator rate were held constant. Compared to the constant flow pattern, the decelerating waveform significantly reduced patient PIP, PaCO2, VD/VT ratio, and alveolar-to-arterial oxygen pressure gradient P(A-a)O2 (46). However, mean Paw was significantly greater, predisposing to adverse hemodynamic effects.
TABLE 102.3 Measurement of Compliance and Airway Resistance |
![]() |

FIGURE 102.14 Differences in peak inflation pressure (PIP) when using a square or decelerating inspiratory flow (VI) waveform. A: An airway pressure (Paw), flow, and volume curve for a typical volume-controlled breath delivered using a square VI waveform. Following breath delivery, an end-inspiratory plateau (EIP) terminates gas flow and allows pressure to equilibrate between the lungs and airway opening; at equilibration, Paw reflects the elastic recoil pressure (ERP) of the respiratory system—–that is, of the lungs and thorax combined. Note the PIP of this breath is nearly 25 cm H2O. B: An airway pressure, flow, and volume curve for the same breath, delivered to the same patient, except using a decelerating VI waveform in this instance. Again, following breath delivery, an EIP allows determination of ERP. Note the PIP of this breath is 8 cm H2O lower than for the square waveform; nevertheless, the measured ERP (18 cm H2O), is exactly the same as that using the square flow pattern; this occurs because a decelerating flow pattern reduces gas flow, to near zero (as during an EIP), before the breath cycles “off” and the EIP begins.
In addition to the potential to improve distribution, decelerating waveforms significantly reduce PIP, especially when contrasted to square (see Fig. 102.14) or accelerating patterns. Some clinicians opt for a decelerating pattern believing the lower PIPs may help protect their patients from ventilator-induced lung injury (VILI). This logic is flawed; the pulmonary edema and lung injury, often seen during mechanical ventilation, are now believed the consequence of excessive volume (volutrauma), rather than excessive pressure (barotrauma) (48,49). Furthermore, the main determinant of volutrauma appears to be end-inspiratory lung volume (the overall lung distension), rather than the FRC (which depends upon PEEP) (19,37). Based on this information, reducing PIP by waveform selection offers no advantage; patients supported using VCV receive the same VT, and therefore overall lung expansion, regardless of waveform.
Inspiratory flow waveforms impact yet another aspect of mechanical ventilation, patient-ventilator synchrony. During any form of patient-triggered mechanical ventilation, the spontaneous inspiratory effort may extend well into mechanical inflation. If at any point spontaneous flow-demand exceeds the preset VI, flow starvation results. Flow starvation distorts pressure patterns and exaggerates WOB. Decelerating flow patterns often provide initial VI sufficient to meet patient demand; however, as the breath proceeds and VI decelerates patient demand may suddenly exceed the available flow and dyssynchrony and flow starvation follow. Management of flow starvation of this nature can, on occasion, be as simple as switching from a decelerating to a constant waveform using a VI set to a value equal to, or greater than, the peak flow used with the decelerating waveform (Fig. 102.15).
Controlled Ventilation
Operational Principles
Mechanical ventilation is indicated when spontaneous ventilation is inadequate or absent. Physiologically, this means the patient is incapable of maintaining acceptable PaCO2 and arterial pH levels. CMV delivers an operator-selected breathing rate, VT, peak VI, and flow pattern; CMV operates completely independent of patient efforts to breathe (Fig. 102.16A). When patients attempt to breathe during CMV, the result can be violent patient-ventilator dyssynchrony. Consequently, patients supported by CMV often require hyperventilation—to blunt the normal stimulus to breathe—heavy sedation, or even pharmacologic paralysis.

FIGURE 102.15 Improving patient-ventilator synchrony using a square instead of decelerating flow waveform, during volume-controlled ventilation (VCV). A: An airway pressure (Paw), flow, and tidal volume (VT) curve for a heavily sedated or very relaxed patient, receiving VCV. B: An airway pressure, flow, and VT curve for the same patient, except the patient is awake, alert, and “fighting the ventilator”; that is, attempting to breathe spontaneously during a machine-delivered breath. Note that both the Paw pressure pattern and VI pattern are distorted by the patient’s effort. Distortions are often exacerbated by the selected flow pattern, particularly the decelerating pattern, which progressively reduces VI, while patient flow demands may remain high. In situations where the patient flow demand exceeds VI from the ventilator, a tremendous additional workload is imposed upon the patient; this predisposes to fatigue and makes managing the patient difficult. C: An airway pressure, flow, and VT curve for the same patient, making the same effort to breathe spontaneously, except the selected VI pattern is switched from decelerating to square. Note that peak VI is nearly identical in each case; yet, by maintaining a high flow longer, a square flow pattern better meets the patient flow demand.
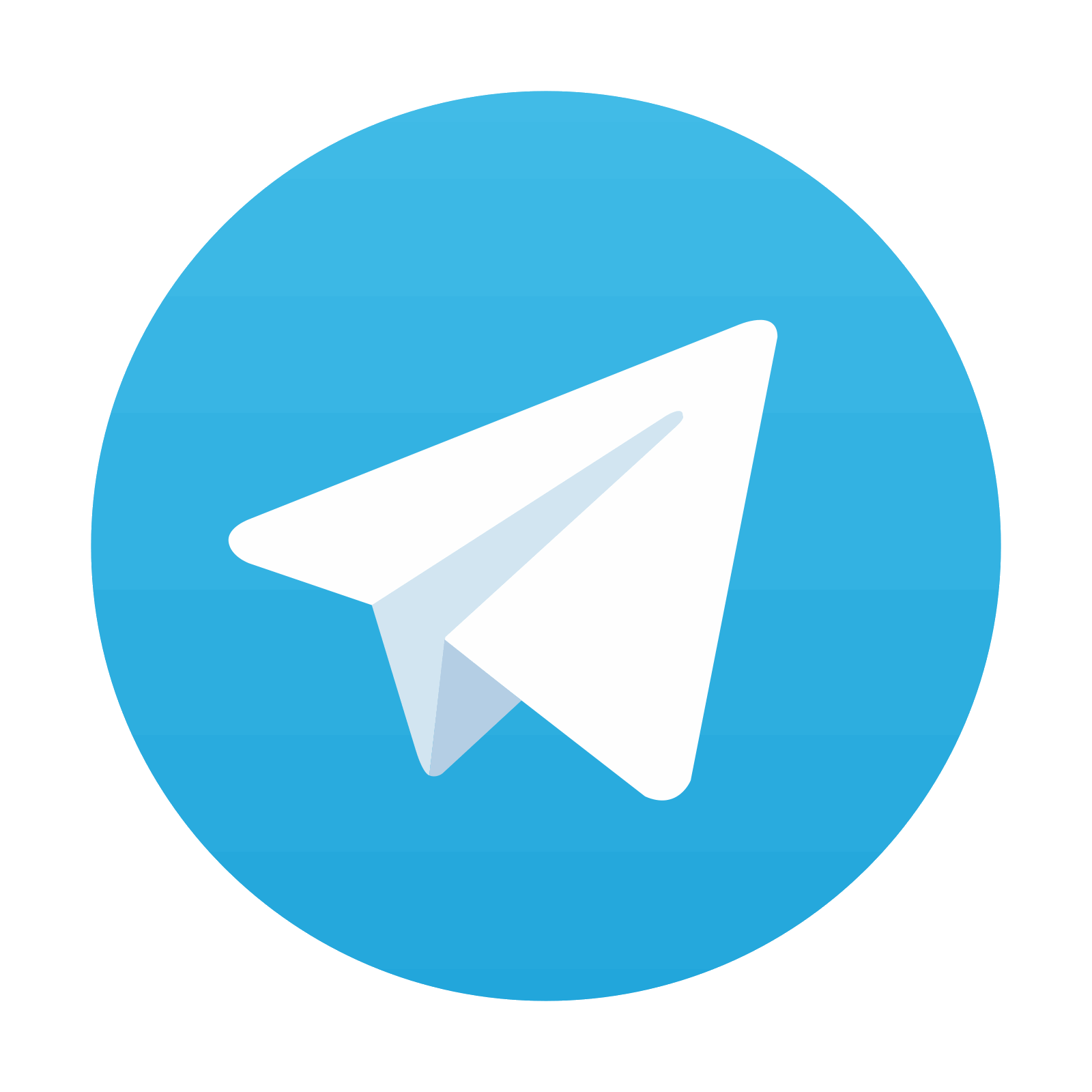
Stay updated, free articles. Join our Telegram channel
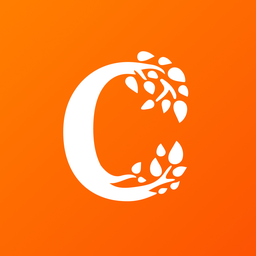
Full access? Get Clinical Tree
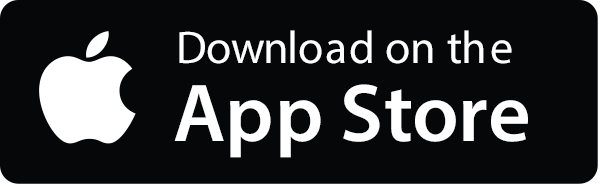
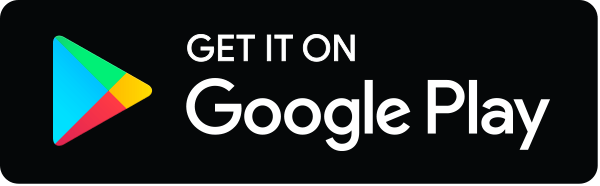