Each ventilator is essentially a controller of pressure, volume, or flow in the equation of motion. The manner in which each variable is controlled, described as the
mode of ventilation,

determines how the ventilator delivers the mechanical breath. In the equation of motion, the form of any of the variables (pressure, volume, and flow are expressed as functions of time) can be predetermined. This principle serves as the theoretical basis for classifying ventilators as
pressure, volume, or
flow controllers (
Fig. 38.1). The necessary and sufficient criteria for determining which variable is controlled are listed in
Table 38.1. It is important to recognize that any ventilator can only directly control one variable—pressure, volume, or flow—at a time. Thus, a ventilator is simply a technology that controls the airway pressure waveform, the inspired volume waveform, or the inspiratory flow waveform, and pressure, volume, and flow are referred to in this context as
control variables.
Control Variables
Control variables relate to the elastic and resistive forces that must be overcome to allow gas delivery to the patient. An initial discussion of the elastic components of the equation of motion as it relates to pressure will help to simplify the explanation. It is known that compliance relates to the change in volume in the lung as a result of a change in pressure. Thus, pressure is related to volume and to the patient’s compliance.
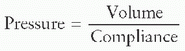
If the clinician sets pressure as the control variable, volume varies directly with the compliance of the respiratory system. Thus, pressure is the independent variable set by the clinician, and volume is the dependent variable determined by the level of pressure. When the pressure pattern is preset by the clinician, the ventilator operates as a pressure controller. The volume becomes a function of compliance, so that a decrease in compliance allows less volume to be delivered for the same pressure. During expiration, the elastic and resistive elements of the respiratory system are passive, and expiratory waveforms are not directly affected by the modes of ventilation or the controller. However, as the respiratory cycle is a set period of time, any change in the inspiratory time can influence expiratory time and, to a certain extent, the expiratory profile.
For the resistive components of the equation of motion,
Pressure = Resistance × Flow
When a ventilator operates as a constant-pressure controller—for example, in pressure-control (
PC) mode, pressure-regulated volume-control (
PRVC) mode, and synchronized, intermittent mandatory ventilation-pressure control (SIMV-PC) mode—pressure is an independent, or controlled, variable (
Table 38.1). The set pressure will be delivered and maintained constant throughout inspiration, independent of what resistive or elastic forces of the respiratory system might be. Even though pressure is constant, the delivered
VT will vary as a function of compliance and resistance, and the flow will also vary exponentially with time.
A waveform from a ventilator operating as a pressure controller is displayed in
Figure 38.2. Under this condition, volume and flow become the dependent variables, and their patterns will depend upon compliance and resistance. When a pressure pattern is preset (constant in a
PC mode), flow-time and volume-time waveforms vary exponentially with time and are a function of compliance and resistance.
It is now clear that flow and resistance are associated only with the resistive components of the equation of motion. The elastic component refers to volume and compliance. Considering the resistive components of the equation of motion,

Thus, if the clinician sets flow as a function of time, pressure then varies with resistance. Flow is the independent variable and pressure is the dependent variable. When a flow pattern is preset, the ventilator operates as a flow controller; pressure is a function of resistance, and the inspiratory pressure-time waveform varies linearly with time. Volume increases linearly with time, although it does not have a direct relation to flow. Based on the equation of motion, volume does have an indirect relationship to flow, as volume is the integral of flow and flow is the derivative of volume. Again, expiration is passive, and the expiratory profile is not directly affected by mode of ventilation, but rather by compliance and resistance, even though the set inspiratory time can influence the expiratory time and, to a certain extent, the expiratory profile.
When a ventilator operates as a constant-flow controller (volume-controlled,
VC, and SIMV-VC modes), flow is the independent variable. Regardless of the resistive or elastic forces of the respiratory system, the set flow will be delivered
and maintained constant throughout inspiration. Pressure and
VT will vary with time, depending on the compliance and resistance.
Waveforms from a ventilator operating as a flow controller are illustrated in
Figure 38.3. Flow is the independent variable (controlled variable); pressure and volume are dependent variables. When a flow pattern is preset (constant in this case), pressure and volume are the dependent variables; they vary linearly with time and are functions of compliance and resistance. The modern ventilator can operate as a
flow controller or as a
pressure controller. As a flow controller, the most common pattern is
constant flow, also referred to as a
square-wave flow pattern. In this mode, the flow increases to a set level that is maintained for the duration of the inspiratory time. The pressure and volume that the patient receives are a function of compliance and resistance. When a ventilator is set as a pressure controller, the observed pressure pattern is constant and results in a
square-wave pressure pattern.
From the equation of motion, it can be stipulated that, with the ventilator operating as a constant-flow controller, the pressure and volume are linear functions of time. The various ventilators are able to deliver various flow patterns. To have flow patterns different from the most common types, which are constant and exponentially decelerating, the ventilator must be controlled by a microprocessor, which performs a series of sequential adjustments dictated by an algorithm to produce various flow patterns, including decelerating ramp, ascending ramp, and sinusoidal. These flow patterns are used in various volume-cycled modes. Again, volume-cycled ventilation is still controlled by flow, and the independent variable set is flow. It is important to note that the decelerating rate of flow is controlled by an algorithm that does not reflect the elastic and resistive elements of the respiratory system. Abnormalities in these elements may result in flow-starvation asynchrony, as the flow from the ventilator may be inadequate to meet patient needs. Not all ventilators can provide novel flow patterns.
Describing the flow and pressure patterns observed in different modes of ventilation is often confusing jargon. To expand further, in
PC ventilation, the pressure pattern is “square,” but flow increases rapidly at the beginning of the inspiratory phase
to generate the set pressure limit, then decays exponentially over the inspiratory time. This flow pattern is described as
decelerating flow. It is said that the major difference between volume and pressure ventilation is based on the square-wave and the decelerating flow patterns observed. In
PC mode, the initial “snap” of high flow to reach the set pressure limit has been thought to be potentially beneficial in opening stiff alveoli in conditions such as acute respiratory distress syndrome (
ARDS) or surfactant deficiency. It has been proposed that a decelerating flow favors better gas exchange and improves distribution of ventilation among lung units with heterogeneous time constants. For this reason, clinicians often choose
PC ventilation in patients with poor compliance, although the true benefit of
PC versus other modes has not been well established in animal or clinical studies.
Volume is most closely associated with the elastic component of the equation of motion. The resistive component of the equation is most dependent on resistance and flow. The

elastic components can be rearranged to display how volume is determined:
Volume = Pressure × Compliance
If the clinician sets volume as a function of time, pressure then varies with compliance. Volume is the independent variable and pressure is the dependent variable.
Theoretically, when a ventilator sets a volume pattern, it operates as a volume controller. However, to be a true volume controller, the ventilator must measure volume directly in order to set the volume pattern. Most ventilators cannot directly measure volume; rather, they calculate volume delivered from flow that occurs over a period of time. Most ventilators use volume as a limiting variable, meaning that inspiration stops when the preselected volume is reached. When inspiration stops at the preset volume value, the ventilator is referred to as
volume cycled, but it is really acting as a flow controller. That is, the ventilator is set to deliver a set
VT at a certain number of breaths per minute and a specified inspiratory time, which determines the patient’s minute ventilation. The ventilator gives a certain flow to meet the set requirements.
Understanding the delivery of a positive-pressure breath to a patient requires an understanding of the relationship of gas delivery to overcoming the resistive and elastic elements of the patient and the ventilator system.
Volume Measurement
The goals of modern mechanical ventilation in infants and children have focused on preventing overdistension of alveoli by limiting
VT, thus reducing volutrauma (
1,
2,
3). Exact knowledge of both inspired and expired gas volumes with a sufficient

level of precision is essential to optimize ventilator settings using lung-protective techniques. During the inflation phase of mechanical ventilation, pressure rises within the ventilator circuit, causing elongation and distension of the tubing and compression of the gas within the circuit. The volume of the gas, which becomes compressed within the ventilator tubing and never reaches the patient, is termed the
compressible volume of the circuit. While in most circumstances, this volume is standard within different sizes of circuits, variation can occur. Knowing the compressible volume within the ventilator circuit is important in determining the actual
VT being delivered to the patient’s lungs. When compression volume is accounted for in determining patient
VT, the resultant volume is termed

the
effective tidal volume (eVT), which means that this is the
VT that reaches the patient’s lungs. eVT can be calculated thus:
eVT = (
VTE ) – [Circuit compensation × (
PImax –
PEEP)]
where
VTE is the expired tidal volume and
PImax is the maximal inspiratory pressure.
The optimal site for monitoring volumes in infants and children is unclear. The inability to accurately measure
VT in a

conventional ventilator is caused by several factors, including (a) difficulty of compensating for volume loss in the ventilator circuit or in the humidifier and (b) changes in temperature, humidification, and secretions, which may also influence the amount of gas that gets delivered from the ventilator to the actual patient. Air leaks around the
ETT itself, especially in small patients with uncuffed tubes, are another source of volume measurement error. Measuring
VT at the proximal airway eliminates most circuit compliance and other dead-space factors. Therefore, it has been recommended that the proximal airway be the only site at which to obtain accurate volume measurements in infants and children (
4). To measure volumes at the proximal airway, a pneumotachograph must be positioned at the patient’s airway opening or at the
ETT. Unfortunately, this technique has disadvantages that are especially apparent in infants and children. A pneumotachograph placed at the proximal
ETT opening creates dead space of its own, which can be detrimental in infants who already have small VTs (
5). In addition, the proximally placed pneumotachograph may impair admittance to the
ETT and airways and make suctioning more difficult. Secretions can also result in contamination of the pneumotachograph and distort observed measurements. Finally, the weight of the pneumotachograph at the proximal end of the
ETT increases its overall weight and may result in an increased risk of extubation.
Given the importance of knowing the true
VT delivered to a patient, it is essential to determine which is the most accurate, safe, and efficient site to monitor. It is also important to understand the reliability of conventional displays of
VT generated by most ventilators. Three clinical studies have compared the ventilator-displayed
VT (measured at the exhalation valve for exhaled
VT displays) with the
VT measured at the
ETT. One group studied 98 ventilated infants and children using the Servo 300
TM ventilator; 70 patients were ventilated with an infant circuit (compliance: 0.61 mL/cm H
2O), and 28 patients were ventilated with a pediatric circuit (compliance: 1.0 mL/cm H
2O) (
6). In the patients with the infant circuit, poor correlation was noted between the expiratory
VT measured with the pneumotachometer and the ventilator-displayed
VT (
R2 = 0.54). Poor correlation (
R2 = 0.58) was also noted between the expiratory
VT measured with the pneumotachometer and the calculated effective
VT. In pediatric circuits, greater correlation occurred between the pneumotachometer-measured expiratory
VT, the ventilator-displayed
VT, and the calculated effective
VT (
R2 = 0.84 and 0.85, respectively).
A second group studied 54 ventilated infants and children using the Servo 300
TM ventilator with a pediatric circuit (compliance: 1.35 mL/cm H
2O) and adult circuit (compliance: 2.4 mL/cm H
2O) (
7). The
VT measured at the
ETT was significantly less than the ventilator-measured tidal
VT and varied between 2% and 91%, with a mean (standard deviation) error of 32% (20%) in 40 children with the pediatric circuit and 18% (6%) in 16 children with the adult circuit. The VTs displayed by the ventilator were also significantly different from the calculated effective
VT (-63.3% to +29.1%), with substantial underestimation of
VT from the
VT displayed or the effective
VT in many patients with small VTs. The third group studied 30 ventilated infants, 1-23 months old, on Servo 300. ventilators with neonatal (compliance: 0.63 mL/cm H
2O) and pediatric (compliance: 1.13 mL/cm H
2O) circuits (
8). This study demonstrated that the expiratory
VT measured at the
ETT was less than the expiratory
VT displayed by the ventilator. When the ventilator was in the
VC mode, the median difference was -36% (range: -5% to -2%), while the median was -35% (range: -6% to -60%) in the
PC mode. The calculated
effective
VT was not statistically different from the ventilator-measured
VT in
PC mode; however, individual differences were large (range: -26% to +52%). The calculated effective
VT was less than the
VT measured at the
ETT in the
VC mode, with large individual differences (median: -23%, range: -48% to +21%). All of these clinical studies have recommended that the
VT should be measured at the
ETT in infants and small children. As stated previously, the effective
VT can be calculated as the ventilator-measured expired
VT. However, this method fails to take into account volume lost internally in the ventilator. Manufacturers have attempted to compensate for these volume losses by measuring compression volume loss in the system. The compliance factor can be calculated as
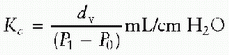
where Kc is the compliance factor, dv is the total integrated volume, and P0 and P1 are the start and target pressures.
Data demonstrate good agreement in volume measured at the ventilator when compensation adjustment is on, as compared to volume measured at the proximal airway (
9). The use of circuit-compliance compensation improved the agreement between the volume measured by the ventilator in an animal trial; pediatric pigs had improved agreement between the two volume methods attributable to circuit-compliance compensation (with circuit-compliance compensation “on,” 0.97; with circuit-compliance compensation “off,” 0.88;
p = 0.027). It is essential for the clinician to understand the accuracy of the delivery of
VT to their patients, especially in patients with small volumes, such as infants.
In a clinical study of 68 ventilated pediatric patients aged between 2 days and 18 years, the principal observation was that, when compression volume was compensated for by a computer algorithm, a negative bias occurred. Displayed volume was lower than that set by the clinician, but agreement was good when using compensation, with a concordance correlation between 0.90 and 0.98. Accuracy, expressed as percent difference, improved only in older patients (
10). A limitation of this study, as well as previous studies that utilized an airway sensor or pneumotachograph as the reference value of volume, is the short time frame in which volumes were measured. A potential limitation of the airway sensor is the increased opportunity for the sensor to be contaminated by airway secretions and foreign substances, as compared to a pneumotachograph located within the ventilator. The potential for contamination, and thus degradation of the accuracy of the signal, of an airway sensor increases with increased length of time that the sensor is in place. In all of these studies, the airway sensor was only in place for a limited period. Caution must be used in extrapolating measurements from previously cited studies when the airway sensor is not left in place over a prolonged period.
When examining volume inaccuracies, the first source of error would be loss of volume within the ventilator circuit resulting from compression volume of the circuit. In the inflation phase, pressure rises within the ventilator circuit causing elongation and distention of the tubing and compression of the gas within the circuit. The volume stored in the circuit never reaches the patient but is instead released through the exhalation valve and is measured with the exhaled gases from the patient. The volume within the ventilator, circuit, and humidifier determines the magnitude of compression volume. The volume loss can theoretically be affected by a number of factors, including temperature and humidity of the circuit, and patient factors such as changes in the patient’s compliance and resistance. In the above-mentioned study, it was found that the reported differences in the measured compliance of the circuit by the manufacturer and those measured by the ventilator with differences were between 37% and 65% for the infant circuit and between 13% and 23% for the adult circuit. Because this factor is collected at startup of the ventilator and includes any compression volume in the ventilator and humidification system, this number will be higher. Subsequently, the compensation measured in the circuit and ventilator system was 51% and 18% higher, respectively, than could be expected from the compression volume calculated using the compliance factor alone. Thus, if only the circuit compliance factor is used, then the volume measurement at expiration, which accounts for both volume of the circuit and volume delivered to the patient, will be higher than the calculated effective volume.
Collectively, studies have shown that the ventilator-displayed
VT, without software compensation for circuit compliance, generally overestimates the true delivered
VT. Conversely, when the circuit-compliance compensation feature

is on, the ventilator-displayed
VT generally underestimates the true delivered
VT.
Trigger Variables
Patient ventilator system interactions can be initiated under two settings: The ventilator can deliver a controlled breath independent of the patient’s desire, or it can be coordinated with the patient’s effort. Ventilators will measure one or more of the variables associated with the equation of motion (e.g., pressure, volume, flow, or time). Inspiration is initiated when one of these variables reaches a preset variable. A patient-triggered breath, sometimes known as interactive ventilation, provides patients with some autonomy to alter breathing patterns in response to their ventilatory demand. Such systems necessitate an interface between the ventilator and the patient to allow for rapid, measured responses from the ventilator to meet patient needs. For such systems to operate, they must sense a signal from the patient and recognize the beginning of inspiration. Second, they must pressurize the system to allow for delivery of the breath to the patient. Finally, the system must recognize the end of inspiration and thus termination of the breath. Ideally, if this interaction could be facilitated by direct interaction between the patient and mechanical ventilator, delays and patient discomfort created by the temporary or relative unavailability of the caregiver at the patient’s bedside could be eliminated.
Initial recognition of the signal from the patient to begin inspiration is commonly referred to as
triggering. Triggering can be subdivided into pretrigger and trigger phases (
12). The pretrigger phase has been defined as the time from the onset of inspiration until triggering occurs. The trigger phase is the time
from triggering until the maximum flow of gas occurs. The most common trigger variables are time and flow.
In
time triggering, the ventilator initiates a breath according to a set frequency independent of the patient’s spontaneous efforts. In flow triggering, the ventilator senses the patient’s inspiratory effort as a change in flow from the baseline flow and begins inspiration independent of the set breath frequency. Ventilator features that affect the trigger phase include the response time of the ventilator and the presence of bias flow.
Bias flow is a continuous delivery of fresh gas circulating through the inspiratory and expiratory limbs of the circuit. Theoretically, bias flow reduces the
WOB by making flow available to satisfy the earliest demand of the patient during inspiration, before the flow is initiated during the pretrigger phase. Increased patient effort to trigger the ventilator and delayed response of the ventilator to the patient’s effort can be translated directly into increased
WOB.
Current ventilator designs have improved patient-ventilator interactions by improving both the signal sensed by the ventilator and the response time of the ventilator. Today, all ventilators have the capability to utilize a flow signal as the trigger signal from the patient to the ventilator. Flow triggering has the advantage of allowing the patient to trigger the ventilator with less effort, and it has a faster response time (
13). The process of creating somewhat seemingly small amounts of negative pressure in pressure-triggered breaths is made increasingly more difficult when the patient has a smaller
ETT and/or in the presence of
PEEPi.
Theoretically, patient-triggered ventilation could be improved if a signal could be acquired from the patient that represented the earliest attempt by the patient to acquire a breath and if that signal could represent the amount of effort or drive from the patient for that breath. This approach is represented in what has been termed
neurally adjusted ventilatory
assist (
NAVA) (
14). The
NAVA approach to mechanical ventilation is based on the acquisition of the patient’s neural respiratory output as it is transmitted through the phrenic nerve to the diaphragm. This signal is acquired via an esophageal catheter with an imbedded series of electrodes that capture the electrical activity signal of the diaphragm, known as
Edi.
NAVA responds by providing the requested level of ventilatory support to the patient from the
Edi. The advantage of this system is the ability to acquire the patient’s desire to trigger the ventilator quickly and to offer feedback between patient effort and ventilator output. At this writing,
NAVA is being investigated in clinical trials in Europe in neonatal, pediatric, and adult patients.
Work of Breathing
Work is equal to the force applied to an object multiplied by the distance the object travels. That is, work = force × distance, or W = F × D. If work is applied into the three dimensions of the respiratory system, work becomes the pressure applied to yield a change in the volume of the system and can be expressed as:
where
P is the integral of the pressure across the respiratory system as a function of volume, and d
v is the change in the volume of the respiratory system.
The concept of work associated with the functioning of the respiratory system has been known since the seminal analysis of Otis et al. (
15). They elucidated that several forces were encountered while breathing, including the elastic forces of the chest wall and lungs, viscous and turbulent resistance of air, nonelastic tissue impedance, and inertia. Basically, motion requires work. Work is performed when pressure changes the volume of the respiratory system and is the product of pressure and volume integrated over time with respect to volume. Work is performed on the respiratory system by externally applied pressures from the ventilator via positive pressure, respiratory muscles, or both, as the lungs expand and contract. To achieve normal ventilation, the body performs work (
WOB) to overcome the elastic and frictional resistance of the lungs and chest wall. Total work of breathing (
WOBT) is the sum of elastic work (
WOBE) and resistive work (
WOBR). Elastic
WOB represents physiologic work to expand the lungs and chest wall. Resistive
WOB is considered a measure of imposed
WOB and includes work caused by the breathing apparatus, such as the
ETT, breathing circuit, and ventilator demand-flow system. Artificial airways and physiologic resistive work of the airways are responsible for a large part of the imposed resistive work, with the mechanical ventilator also contributing some portion of resistive work (
16).
Clinicians have long recognized that increased
WOB occurs in patients being weaned from prolonged mechanical ventilation, when the patient begins to breathe spontaneously and take on more of the
WOB. Patient-related factors, equipment factors, and decision making affect weaning of patients from mechanical ventilation and, thus,
WOB. Equipment factors relate to the ability of the mechanical ventilator to meet the needs of the patient. It has been demonstrated in a lung model that the amount of
WOB varies according to the device utilized (
17). These equipment factors have an increased significance in patients with poor pulmonary reserve or high airway resistance, where the
WOB associated with the equipment is increased (
18,
19). These factors also have an increased significance in pediatric patients, in whom equipment is often associated with increased
WOB (
20).