There are many features of the administration of general anesthesia that differ between children and adults. In this chapter, we discuss those differences, with an emphasis on anesthetic medications and ventilation strategies. We also review the importance of racial disparities in pediatric anesthesia, surgical site infection prophylaxis, and the possibility of neurotoxicity of anesthetics to the developing brain.
In the United States, most children that require general anesthesia undergo inhalational induction by face mask with intravenous (IV) catheter placement after loss of consciousness ( Fig. 19.1 ). Most hospitals have taken pride in their “ouch-less” environment, and thus, parents expect that their children will not undergo any painful injections while awake. This strategy is entirely optional, as some children prefer IV insertion while awake instead of the uncomfortable feeling of having a noxious mask over their face.

Not all (healthy) children that are administered sevoflurane require IV access during general anesthesia. Two common examples are myringotomy for ear tube insertion and computed tomography (CT) scan, mainly because they are very brief procedures.
For difficult IV placement in anesthetized children, it has become standard practice to use ultrasound guidance. The use of ultrasound has higher first attempt success rates and greater overall success of cannulation than without ultrasound. The short axis out-of-plane technique together with dynamic needle position, where the needle tip is followed as the ultrasound probe is moved proximally, may further increase the success rate of peripheral IV placement in small children.
Sevoflurane
Sevoflurane is the anesthetic agent of choice for inhalational induction of general anesthesia in children because of its relatively low pungency and its lower blood-gas solubility coefficient (0.63) that speeds loss of consciousness.
The minimum alveolar concentration (MAC) of sevoflurane is 3.2% in neonates and infants up to 6 months of age; the MAC of sevoflurane peaks at 3 months of age. In school-aged children, MAC is 2.5%; the concentration that prevents 95% of children from moving in response to a surgical stimulus (ED95) is 2.9%. When 60% nitrous oxide (N 2 O) is added to sevoflurane, the MAC is lowered to 2.0%. Summary graphs of MAC according to age have been published.
Induction of general anesthesia with sevoflurane may be accomplished in several different ways. In some cases, the vaporizer is set to the maximal 8% setting from the start. Whether or not this is combined with N 2 O, most children will lose consciousness within 5 to 10 breaths.
Most children develop tachycardia during inhalational induction with sevoflurane. Twenty percent of children will develop a nodal rhythm and infants less than 6 months of age may demonstrate lengthening of the QT interval that continues into the postoperative period. These changes, however, do not result in adverse clinical manifestations. Sevoflurane may cause a dose-dependent bradycardia and hypotension in some children, especially those with trisomy 21; pretreatment with IV glycopyrrolate or oral/intramuscular (IM) atropine may be considered in this population.
During the early stages of sevoflurane induction, a peculiar type of agitation may be observed, mainly in teenagers. It consists of muscular rigidity and generalized tonic-clonic or myoclonic movements, and can worry some practitioners that it is the onset of malignant hyperthermia (it is not). It may be caused by electrical seizure activity , especially when concentrations exceed 4.5%. This effect is accentuated with hyperventilation, and is suppressed by N 2 O and opioids. These stimulatory effects of the central nervous system are not associated with postoperative sequelae.
Single-Breath Induction Technique
The single-breath induction technique ( ) will considerably speed the loss of consciousness in older, cooperative children. It is performed by priming the anesthesia circuit and ventilation bag with 70% N 2 O and 8% sevoflurane. The distal end of the breathing circuit is sealed off to prevent leakage of sevoflurane into the operating room (OR) environment. The child is instructed to first blow all the air out of their lungs (it is helpful to practice this technique with the child before entering the OR). At the very end of the child’s exhalation, the facemask is placed over the child’s mouth and nose, and the child is instructed to take “the biggest breath of their life.” This technique invariably results in loss of consciousness soon after the vital capacity breath.
Steal Induction
When a child is asleep upon entering the OR, a “steal” induction may be performed. The anesthesia breathing circuit is primed with N 2 O and sevoflurane in a similar fashion as that done for the single-breath technique. The child is not touched or moved to the OR table and no monitors are applied. The face mask is moved progressively closer to the child’s face without touching it. Once consciousness is lost, the child is moved to the OR table and monitors are applied. Proponents of this technique favor its atraumatic nature and the child’s lack of awareness of the OR environment. Opponents of this technique fear that a child could suffer psychological harm if they awaken from a painful procedure without adequate psychological preparation.
Nitrous Oxide
N 2 O can be used as an adjunct to inhalational induction because of its ability to reduce the MAC of inhaled agents and to speed the onset of unconsciousness via the second gas effect. It is discontinued when the IV catheter is inserted.
N 2 O has been associated with myoclonic movements and even generalized seizures in some unusual cases. Because N 2 O decreases the activity of two vitamin B 12 enzymes, methionine synthetase and thymidylate synthetase, its use has been implicated in the exacerbation of vitamin B 12 deficiency with development of neurologic symptoms in susceptible patients. Therefore, N 2 O should be avoided in children with vitamin B 12 deficiency or a known homozygous MTHFR (methylenetetrahydrofolate reductase gene) mutation. It has also been implicated in causing increased plasma homocysteine concentrations , the clinical significance of which is unknown.
Because N 2 O is equally as flammable as oxygen, it should not be used during procedures that carry risk for airway fire, such as a tonsillectomy (especially when using an uncuffed endotracheal tube, which you should not do anyway; see Chapter 22 ) or laser bronchoscopy, in which the oxygen concentration is lowered as much as possibly safe. Because of these above-mentioned drawbacks of N 2 O, its use has markedly decreased in lieu of using higher concentrations of sevoflurane. Also, N 2 O will diffuse into the endotracheal tube cuff and may cause tracheal edema from overly high cuff pressures if not monitored.
Propofol
IV induction of general anesthesia is reserved for children with established IV access, those with susceptibility to malignant hyperthermia, and those that require a rapid sequence induction (RSI) technique.
Propofol causes immediate loss of consciousness after administration of 3 to 6 mg/kg. Children require larger induction doses than adults because of a larger volume of distribution. Obese children, however, require less than normal-weight children of the same weight and therefore should receive propofol dosed at ideal bodyweight. Maintenance doses are also larger in children because of greater elimination and clearance. Propofol is particularly well suited for use in asthmatics because it blunts airway responses within its clinical dosing range. Propofol usually causes central apnea when administered as a bolus for induction of general anesthesia. If given in titrated doses, however, apnea can be avoided. The apneic dose of propofol is usually less than the dose required to prevent movement in response to a surgical stimulus. Administration of propofol may cause cardiovascular depression in hypovolemic children or those with a preexisting cardiomyopathy and should therefore be avoided or used with extreme caution.
Propofol injection can cause pain at the injection site. Although different methods have been studied to eliminate this pain, the most reliable method is to administer a small volume of 1% or 2% lidocaine while holding pressure proximal to the vein (i.e., a Bier block technique with your hand). Pressure is held for 5 to 10 seconds to assure that the wall of the vein is anesthetized after which the propofol is administered. This method effectively blunts the pain in most children.
During nonpainful medical procedures that require immobility (e.g., radiologic procedures) propofol can be used in moderate infusion doses (150–250 mcg/kg/min) that preserve spontaneous ventilation. For painful procedures (e.g., bone marrow biopsy, lumbar puncture, burn dressing changes) and surgical procedures that require a total intravenous anesthesia (TIVA) technique (e.g., rigid bronchoscopy, malignant hyperthermia-susceptible patient), greater doses of propofol are required to ensure immobility. These larger doses are inevitably associated with central or obstructive apnea. Alternatively, propofol can be combined with an opioid to decrease the total required propofol dose, but assisted ventilation will almost always be required.
Propofol is manufactured from the lipid components of egg and soy. Although these components may contain trace amounts of the residual protein, there have been no documented allergic reactions. Therefore, our policy has been to administer propofol to children with allergy to soy or egg protein, except in the case of documented anaphylaxis to egg or soy, where we practice more conservatively. Over the course of many years doing this, we are not aware of any allergic reactions.
Ketamine
In some cases, ketamine can be used instead of propofol as an induction and maintenance general anesthetic agent. At clinically useful doses (1–2 mg/kg IV), ketamine usually preserves spontaneous ventilation, upper airway patency, and normal cardiovascular function while providing analgesia and amnesia during painful procedures. Ketamine stimulates the sympathetic nervous system, which may cause undesirable increases in blood pressure, intracranial pressure, and intraocular pressure. However, it is useful for induction of anesthesia in patients in which cardiovascular stability is required, such as in the case of trauma or cardiomyopathy. Ketamine is associated with psychomimetic side effects (e.g., hallucinations, nightmares), increased airway secretions, postoperative nausea and vomiting, and delayed awakening. For these reasons, it has largely been replaced by propofol except for brief sedation for painful procedures and intramuscularly (2–4 mg/kg) as a sedative for uncooperative and/or developmentally delayed children.
Etomidate
Etomidate is mainly used in adults with cardiovascular disease and limited cardiovascular reserve. In these patients, it provides a stable induction with limited cardiovascular perturbation. Like ketamine, it may be useful in the traumatized child who is hypovolemic or in a child with a cardiomyopathy and decreased cardiovascular function. The dose range (0.2–0.3 mg/kg) and side effects (e.g., pain on injection, myoclonus, vomiting) appear to be similar to those in adults, including the risk for delayed adrenal suppression. Because of this risk for adrenal suppression, it is rarely used in pediatrics.
Opioids
Opioids are one component of a balanced anesthesia technique, contribute to postoperative analgesia, and attenuate or prevent postoperative emergence delirium or agitation. The choice of opioid depends on the nature and length of the surgical procedure and the expected duration and intensity of postoperative pain. For example, intranasal or intramuscular fentanyl (1–2 μg/kg) is often administered to children undergoing myringotomy and tube placement. IV fentanyl is administered for neurosurgical procedures because of the intense analgesia required for scalp incision and placement of skull tongs, and relatively fewer analgesic requirements during the procedure and postoperatively. Morphine or hydromorphone are administered for urologic and abdominal procedures because of the requirement for a relatively longer duration and intensity of postoperative analgesia.
Opioids are commonly included as a component of a TIVA technique for painful surgical procedures. Fentanyl and its congeners (e.g., alfentanil, sufentanil, and remifentanil) are ideally suited for use in TIVA because of their relatively low context sensitive half-time. Remifentanil possesses the most favorable profile as its termination of action is directly related to its metabolism by tissue and nonspecific plasma esterases. Its effects usually dissipate within 5 to 10 minutes of discontinuing the infusion, regardless of the duration of the infusion.
Remifentanil bolus and infusion doses are higher in infants and young children than in adults, reflecting the larger volume of distribution and increased elimination clearance, respectively. Typically, a bolus dose of 1 to 2 μg/kg will be administered over several minutes, followed by an infusion dose of 0.2 to 1 μg/kg/min. This infusion dose is then titrated up or down to achieve the desired analgesic and hemodynamic effects. The use of intraoperative remifentanil has been associated with development of tolerance and increased postoperative pain.
Historically, pediatric anesthesiologists have had concerns about the increased toxicity profile of opioids in the neonatal population because it is possible that opioids (especially the less lipid-soluble drug morphine) are allowed greater access through the blood-brain barrier in neonates and may result in proportionately greater levels in the brain. Furthermore, neonates have been shown to possess increased pharmacodynamic sensitivity, decreased clearance, and a relatively greater depression of CO 2 response curves to opioids compared with older subjects. These maturational changes appear to be most pronounced for morphine in comparison with fentanyl and its analogs. Opioids, like all types of medications administered to neonates, possess substantial interindividual variation in their pharmacokinetic and pharmacodynamic properties; thus, they should be titrated to effect while carefully observing for efficacy and side effects.
Dexmedetomidine
Dexmedetomidine is an α 2 -adrenoceptor agonist that is more receptor specific than clonidine. It has been primarily used in pediatric anesthesia to accomplish sedation for medical procedures or postoperative ventilation. Preoperatively, intranasal dexmedetomidine has been used for anxiolysis and sedation before induction of anesthesia. A 2 µg/kg intranasal dose produced faster onset of sedation without significant difference in induction, emergence and recovery compared with 0.5 mg/kg of oral midazolam. Intraoperatively, dexmedetomidine has been used as an adjunct in airway procedures and in both sleep endoscopy and airway imaging. In neurosurgery, it may be used by itself for tumor and epileptic seizure foci resection and mapping. Perioperatively, compared with placebo, dexmedetomidine at a dose of 0.5 to 1 µg/kg can decrease the incidence of emergence delirium by 10-fold. Dexmedetomidine administration is also associated with decreased intraoperative and postoperative opioid use.
Dexmedetomidine administration has been associated with hypotension and bradycardia (up to 30% decrease from baseline). Therefore, it should be avoided in children receiving digoxin, beta blockers, or agents that predispose to bradycardia or hypotension. Administration of an anticholinergic agent after dexmedetomidine has been associated with severe hypertension.
Neuromuscular Blockers
Neuromuscular blockers are often administered to facilitate endotracheal intubation and may be continued intraoperatively to optimize surgical conditions and positive pressure ventilation.
There are developmental physiologic differences that influence the pharmacology of neuromuscular blockers ( Table 19.1 ). During growth in early childhood, the increase in muscle volume increases the number of neuromuscular receptors. Conduction velocity and myelination increase during development and the rate of acetylcholine release progressively increases. Taken together, these are manifested clinically as a greater pharmacodynamic sensitivity to neuromuscular blockers in infants and young children (i.e., neuromuscular blockers are more potent in these age groups). Indeed, unanesthetized newborns demonstrate significant fade when applying 50-Hz tetanic stimulation, which suggests that their acetylcholine supply can be readily exhausted.
Infants | Children | Adults | |
---|---|---|---|
Atracurium | 0.24 | 0.33 | 0.21 |
Cisatracurium | 0.06 | 0.06 | 0.05 |
Pancuronium | 0.065 | 0.10 | 0.07 |
Rocuronium | 0.25 | 0.40 | 0.35 |
Vecuronium | 0.05 | 0.08 | 0.04 |
Succinylcholine | 0.61 | 0.35 | 0.29 |
In practice, three (or more) times the ED 95 is administered to assure rapid paralysis and account for pharmacokinetic and pharmacodynamic differences between individual patients. Nondepolarizing neuromuscular blockers are more potent (i.e., longer duration at similar doses) in infants than in children and less potent in children than adults.
Because all neuromuscular blockers are water-soluble and younger children are known to be composed of a relatively greater volume of body water, the volume of distribution for these drugs is larger in younger children compared with older children and adults. Clinically, this translates to a higher bolus dose required to achieve a given plasma level.
Because neonates and small infants demonstrate enhanced sensitivity to neuromuscular blockers, a lower plasma concentration is required to maintain paralysis so the bolus maintenance dose is the same in a mg/kg basis as it is for adults. Neonates and small infants will also demonstrate a prolonged duration of action because of the larger volume of distribution and the decreased liver function in the newborn period. Because of this latter characteristic, the aminosteroidal relaxants, such as vecuronium and rocuronium, which rely on liver metabolism for their termination of effect, become long-acting agents, often exceeding 60 minutes or more in neonates.
Succinylcholine
Succinylcholine is the fastest acting agent with the shortest duration of action. Despite its many drawbacks in children, it remains the agent of choice for rapid sequence induction and for life-threatening upper airway obstruction, such as laryngospasm. In the latter situation, it can be given IM before establishment of IV access during inhalational induction.
Because succinylcholine is water-soluble, the IV bolus dose required in infants and young children (2 mg/kg) is larger than the dose for older children and adults (1 mg/kg). An intramuscular dose of 4 mg/kg will have an onset of action within a minute, a maximum onset of blockade in 3 to 4 minutes, and duration of action of approximately 20 minutes. In children, its duration of effect is less than in adults because its elimination clearance is more rapid.
There are a number of important side effects and complications of succinylcholine that include vagal-mediated bradycardia, junctional rhythms, or sinus arrest especially after the second dose within a short period of time. Some pediatric anesthesiologists administer an anticholinergic agent before succinylcholine to prevent bradycardia. IV atropine (0.02 mg/kg) and glycopyrrolate (0.01 mg/kg) are equally effective in attenuating succinylcholine-induced bradycardia.
Because of the low overall muscle mass in young children, fasciculations and postoperative muscle pain after administration of succinylcholine are unusual. However, muscle pain, rhabdomyolysis, and myoglobinuria may be observed when repeat doses are given. When these occur after just one dose of succinylcholine, the child should be investigated for an occult myopathy.
Because succinylcholine-induced paralysis causes muscle contraction by simulating the action of acetylcholine, potassium is released from the intracellular space and a transient and clinically insignificant increase in the plasma potassium level normally occurs. However, in patients with muscle atrophy or disorders associated with up-regulation of extrajunctional acetylcholine receptors (e.g., burns), an exaggerated potassium release will occur. The hyperkalemia that develops can lead to arrhythmias, cardiac arrest, and death.
An important publication in 1997 by Larach et al. alerted the anesthesiology community to a number of cases of succinylcholine-induced life-threatening hyperkalemia in young boys with undiagnosed Duchenne muscular dystrophy. As a result, anesthesiologists generally avoid succinylcholine for routine, elective neuromuscular blockade. But because succinylcholine is still indicated in some situations, all anesthesiologists should be aware that hyperkalemia is manifested as peaked T waves on the electrocardiogram (ECG), which may develop into a wide complex tachycardia, ventricular fibrillation, and asystole. If this occurs, one should not wait to confirm with a blood-potassium level, but should begin immediate treatment with calcium chloride (5–10 mg/kg) or calcium gluconate (50–100 mg/kg) followed by a beta-agonist such as albuterol, insulin and glucose, sodium polystyrene (via orogastric tube), furosemide, and dialysis if clinically indicated.
Succinylcholine-induced hyperkalemia can occur in patients with any type of disease associated with muscle atrophy, especially when progressive (e.g., Duchenne muscular dystrophy) or after an acute muscle injury or burn. Children with cerebral palsy or meningomyelocele demonstrate a normal potassium release after succinylcholine; thus, the use of succinylcholine in these patient groups is not contraindicated.
In Larach’s important description of 25 children who had a cardiac arrest within 24 hours of receiving an anesthetic, 20 were previously healthy. The presenting cardiac symptoms included wide complex bradycardia, ventricular tachycardia with hypotension, ventricular fibrillation, and asystole. Hyperkalemia occurred (mean peak serum K + 7.4 ± 2.8 mmol/L [median 7.5, range 3.5–14.8]) in 13 of 18 patients in whom potassium level was measured during the cardiac arrest. Eight of the 13 patients with hyperkalemia received both succinylcholine and potent inhaled anesthetics, while one patient received succinylcholine alone, and four received inhaled anesthetics without succinylcholine. Fifteen patients survived, most with eventual return of baseline neurologic function. Of the 13 patients with hyperkalemia, 8 were eventually shown to have an occult myopathy that was previously unknown to the family or anesthesia team. Anesthesiologists should always rule out an occult myopathy in children preoperatively by inquiring about abnormal muscle weakness or delayed (or lost) motor milestones. The failure of a child to walk unassisted by 15 months of age should prompt further evaluation. Clinical features of Duchenne muscular dystrophy include calf muscle hypertrophy, toe walking, and a waddling gait. An elevated creatine kinase level may indicate an occult myopathy.
Based largely on this report, the Food and Drug Administration (FDA) asked the manufacturer to place a black box warning in the package insert of succinylcholine. The report and the black box warning led to the precipitous decline of elective use of succinylcholine in children.
Succinylcholine Black-Box Warning
“It is recommended that the use of succinylcholine in children should be reserved for emergency intubation or instances where immediate securing of the airway is necessary, e.g., laryngospasm, difficult airway, full stomach, or for intramuscular use when a suitable vein is inaccessible.”
Along with inhaled anesthetic agents, succinylcholine may trigger malignant hyperthermia (MH) in susceptible patients. When succinylcholine was used routinely, the incidence of MH was estimated to be 1 in 15,000 children. In retrospect, many of these cases were probably rhabdomyolysis-induced hyperkalemia rather than MH, but the possibility of triggering MH is another reason that succinylcholine is no longer used in children electively.
On occasion (1% or more in some studies), masseter muscle rigidity (MMR) may occur after succinylcholine administration. It ranges from a mild difficulty with mouth opening to the complete inability to open the mouth (“jaws of steel”). This may represent a normal response to succinylcholine, especially if underdosed. When severe, or accompanied by generalized rigidity, it can represent an early sign of MH.
If severe MMR occurs, all volatile anesthetics should be discontinued and a nontriggering technique with TIVA administered. Creatine kinase levels should be obtained along with electrolytes and blood gas analysis. The patient should be observed closely for signs and symptoms suggestive of an acute MH crisis. Treatment with dantrolene is not indicated unless there are definitive signs of MH.
Nondepolarizing Neuromuscular Blockers
The nondepolarizing neuromuscular blockers are divided into two major categories based on structure and mode of elimination. The benzylisoquinoliniums (e.g., atracurium, cisatracurium) rely on metabolism by Hofmann degradation and ester hydrolysis by nonspecific plasma esterases for their termination of action. The aminosteroids (e.g., vecuronium, rocuronium) are metabolized in the liver to inactive products that are eliminated by the kidney. A major advantage of the aminosteroids is their efficacy after intramuscular administration.
Atracurium, 0.5 mg/kg, usually produces reliable intubating conditions within 90 seconds after administration. Its duration of action is approximately 30 to 40 minutes but is usually reversible within 20 minutes after administration. Because its metabolism does not rely on hepatic function, its duration of action is predictable, even in neonates. Large doses of atracurium may result in histamine release that is manifested as a macular rash, and rarely, bronchospasm and hypotension.
Cisatracurium is a cis isomer of atracurium and possesses a similar clinical and pharmacokinetic profile except for its increased potency and lack of histamine release. Its onset to maximum block is slower than atracurium. Typically, a dose of 0.15 to 0.2 mg/kg will provide reliable intubating conditions within 2 minutes. Clinical studies in children indicate that administration of cisatracurium is associated with a slightly longer and less predictable duration of action than atracurium; compared with other agents, cisatracurium is still relatively predictable given its metabolism via Hofmann elimination.
Vecuronium, 0.1 mg/kg, usually provides reliable intubating conditions within 2 minutes. Its duration of action is approximately 40 to 75 minutes but is usually reversible within 30 minutes of administration. School-aged children require relatively more vecuronium to achieve a desired effect and recover faster than infants and adults. Because its termination depends on liver metabolism, the duration of action can be prolonged in neonates and small infants or in patients with hepatic dysfunction. Vecuronium administration is associated with bradycardia when given in combination with fentanyl or one of its analogs. However, vecuronium is not associated with histamine release or bronchospasm.
It is possible to use vecuronium for rapid sequence induction. At a dose of 0.4 mg/kg (four times a typical intubating dose) reliable intubation conditions can be achieved within 1 minute but at the expense of a prolonged duration of action: reversibility may not be possible for more than 90 minutes or shorter with the administration of sugammadex. This large dose can be decreased by also administering an opioid.
Rocuronium has a similar clinical profile as vecuronium and atracurium. Its main advantage is its ability to be given in higher doses (1.2–1.6 mg/kg) to achieve reliable intubating conditions within 1 minute. These higher doses are not associated with the prolonged duration of action seen with vecuronium; reversibility with neostigmine is usually possible within 45 minutes, or much shorter with administration of sugammadex. Therefore, rocuronium is often preferred to succinylcholine for rapid sequence induction in children.
When administered intramuscularly (1.0 mg/kg for infants and 1.8 mg/kg for children), rocuronium provides reliable intubating conditions within 3 minutes at the expense of a prolonged duration of action that may exceed an hour. A deltoid injection provides more reliable plasma levels than a quadriceps injection.
Ventilating Children During General Anesthesia
Pediatric patients undergoing surgery often require mechanical ventilation, especially if intubated. Like most aspects of pediatric care, the tolerance for error is smaller than in adults because small variations can be a significant percentage of the intended therapy. With regard to mechanical ventilation, the most important consideration is that small changes in delivered tidal volume can be a significant percentage of the intended tidal volume. Consequences of unintended variation in tidal volume can be hypoventilation with hypercarbia and atelectasis, or excessive tidal volume with attendant hypocarbia and barotrauma or volutrauma.
On most operating room ventilators, there are both volume and pressure-controlled ventilation modes. With pressure-controlled ventilation, a peak inspiratory pressure is selected (in children with healthy lungs usually between 15 and 18 cmH 2 O), and the rate is set to achieve the desired carbon dioxide concentration. One should recognize that volume is not guaranteed in pressure mode and will vary with changes in lung compliance. Pressure mode is most useful when changes in lung compliance are not likely although tidal volume monitoring is important. It is useful to set a low tidal volume or minute ventilation alarm close to the desired value so that one will be aware of changes in lung compliance that reduce tidal volume.
Pressure mode has historically been preferred in children because earlier generations of anesthesia ventilators could not deliver small tidal volumes accurately in volume mode. Current approaches to mechanical ventilation emphasize limiting tidal volume to 5 to 7 mL/kg of ideal bodyweight to reduce the potential for ventilator-induced lung injury. Given the desire to control tidal volume, volume controlled (targeted) modes of ventilation make it easier to implement a lung protective ventilation strategy. Modern anesthesia ventilators designed to compensate for the compliance of the breathing circuit are capable of delivering even small tidal volumes accurately. Therefore, volume-controlled ventilation is a very reasonable choice even for small children when using one of these anesthesia ventilators. When using volume-controlled ventilation, a set tidal volume is selected (usually 5–7 mL/kg) and the rate is set to achieve the desired carbon dioxide concentration. Pressure will vary with changes in lung compliance, so it is useful to set an inspiratory pressure limit to prevent excessive pressures because of transient decreases in effective lung compliance like coughing or surgical retraction. Regardless of whether pressure or volume controlled ventilation is used, alterations can be made to the overall ventilation, including changes in the inspiratory to expiratory time ratios and the addition of positive end-expiratory pressure (PEEP) (in healthy children we usually use a PEEP of at least 4–5 cmH 2 O to prevent loss of FRC) to ensure adequate gas exchange.
It is important to monitor inspiratory pressure, tidal volume and end-tidal CO 2 to assess the interaction of the ventilator with the patient. Because arterial blood gas information is not routinely available, assessing oxygenation using SpO 2 at an inspiratory oxygen concentration of 25% or less will help guide adjustments to PEEP and tidal volume and the need for recruitment maneuvers. Tidal volumes of 7 mL/kg are adequate for most patients and will result in an end-tidal CO 2 measurement that will approximate the arterial CO 2 value.
There are different opinions about the value of neuromuscular blockade on ventilatory function during general anesthesia. One study demonstrated a decrease of functional residual capacity (FRC) and ventilation homogeneity after onset of paralysis. In another study of spontaneously breathing children, the performance of a recruitment maneuver to 15 cmH 2 O resulted in less atelectasis (measured by MRI) than a control group of children that received only CPAP of 5 cmH 2 O.
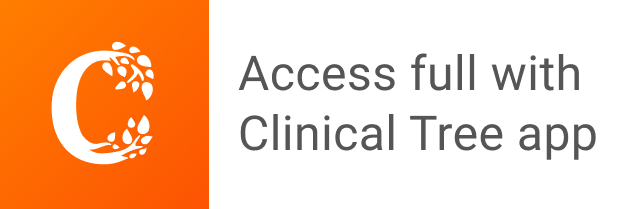