Abstract
Magnetic resonance imaging (MRI) has earned a prominent and revolutionary role in diagnostic imaging by allowing high-resolution image acquisition without harmful ionizing radiations. MRI has also gained recognition as an indispensible therapeutic adjunct in minimally invasive neurosurgical procedures by allowing precise targeting of lesions in patients with tumors or movement disorders. Newer applications such as functional MRI and magnetic resonance spectroscopy allow visualization and mapping of activity of task-specific neuronal networks, which has significant implications across a number of specialties including functional neurosurgery and psychiatry. The unique hazards of strong magnetic and radiofrequency fields, the confined space and prolonged imaging times of MRI pose unique challenges and significant limitations to patients and caregivers alike. Strict regulations serve to enhance the safe delivery of MRI. The provision of MRI for patients with implantable electronic devices such as pacemakers remains a particular area of concern and focus of research and guideline development. Anesthesiologists must have a thorough understanding of the unique aspects of anesthetic management in the MRI environment.
Keywords
Anesthetic implications, Hazards, Magnetic resonance imaging, MRI suites, Neuroradiology
Outline
Introduction: The Road From X-Ray to Magnetic Resonance Imaging 519
Principles of Nuclear Magnetic Resonance and Magnetic Resonance Imaging 521
Various Types of Signals Recorded 522
Hazards Related to Magnetic Resonance Imaging 524
Hazards Related to High-Power Magnetic Field 524
Hazards Related to Radiofrequency Field 525
Image Acquisition–Related Hazards: Prolonged Imaging Time, Noise, Heating 525
Contrast Agent–Related Hazards 525
Hazards in Patients With Cardiac Implantable Electronic Devices and Other Implantable Devices 526
Magnetic Resonance Imaging Safety: General Considerations 526
Magnetic Resonance Imaging Zones 526
Patient and Personnel Screening 527
Object and Device Screening 527
Magnetic Resonance Imaging Safety: Management of Cardiac Implantable Electronic Devices and Other Implantable Devices 527
Anesthesia for Magnetic Resonance Imaging 530
Anesthetic Management and American Society of Anesthesiologists’ Practice Advisory on Anesthetic Care for Magnetic Resonance Imaging 530
Research Applications/Emerging Clinical Applications of Magnetic Resonance Imaging 531
Functional Magnetic Resonance Imaging 531
References 532
Introduction: The Road From X-Ray to Magnetic Resonance Imaging
The field of radiology and diagnostic imaging (as it is referred to now) has progressed through some revolutionary scientific innovations in the past century. With every breakthrough, while the diagnostic capabilities improved dramatically the practice of medicine also benefited significantly. The major imaging techniques that were invented/developed in the past century started with the plain X-ray, followed by the cerebral angiogram and several imaging techniques with contrast dye, which can be imaged with X-rays. Then came ultrasound-based imaging techniques. Ultrasound became popular because it is noninvasive, unlike X-ray there is no radiation hazard, and above all, it is portable and can be performed at the patients’ bedside. After the ultrasound, the next major invention (and a revolutionary one) was computerized axial tomography (CAT). CAT scan is based on differential absorption of X-rays by tissues. Based on differential absorption of X-rays, various tissues like brain matter, cerebrospinal fluid (CSF), blood, and bone could be identified and imaged with precision. For the first time in the history of medical diagnostic imaging, brain (which was considered to be a black box, difficult to visualize) could be clearly imaged and many intracranial pathologies like brain tumor, intracranial bleeding, and brain contusion could be diagnosed based on imaging. At this point, tomography and the available computing technology where incorporated in imaging technology, which was a great advancement in imaging technology. With computing techniques, it is now possible to acquire, analyze, store, and interpret large amount of data and create images based on that. After CAT scan the next major invention in medical imaging was the introduction of the magnetic resonance imaging (MRI). MRI was a major revolution in its own way because while this technique improved the spatial resolution of imaging significantly, unlike the other available imaging techniques in the 1980s (developed in the preceding 80+ years) it is not an X-ray-based technique. It is based on magnetic resonance (MR) property of H + ions (which brought its own challenges but minus the hazards of X-rays). At this point the terminology “Department of Radiology” also had to be modified to “Department of Radiology and Diagnostic Imaging.”
As alluded to earlier at every stage with improved imaging techniques in the practice of medicine, quality of medical care also improved in a great way. The scientific significance of these innovations in medical diagnostic imaging was recognized by several Nobel Prize awards. Prof. Allan Cormack and Sir Godfrey Hounsfield were awarded the Nobel Prize in medicine and physiology in 1979 for their work related to CAT scan. In 2003, Prof Paul Lauterbur and Sir Peter Mansfield were awarded the Nobel Prize for their contribution to the invention of MRI. Prior to this three more Nobel Prizes have been awarded in the field of MR (Isidor Rabi in 1945 followed by Felix Bloch and Edward Purcell in 1952). This signifies that MRI invention was really a ground-breaking scientific innovation, in addition to its significance in medical diagnostic imaging as a whole.
Although MRI and CAT scan imaging techniques have impacted and brought about significant advancement in all fields of medicine, the field of neuroscience seems to have benefited the most. Prior to the CAT scan and MRI era, the interior of the brain, which has been considered to be a black box all along, could never be visualized or imaged. Only indirect images of the brain were possible before CAT and MRI (looking at the shift in vascular tree and midline structures caused by a space-occupying lesion in the brain or within the cranium). CAT scan and MRI permitted high-resolution images of the interior of the brain comparable to illustrations available in standard medical text books. As a result, pathological lesions in the brain like brain tumors, intracranial bleeding, brain contusions, and stroke could be visualized and diagnosed early, which in turn resulted in earlier treatment and better outcome for the patients. The first MRI scanner was installed in the United States in 1982. In 1985, the US Food and Drug Administration (FDA) approved scanners for clinical applications, and this allowed physicians to prescribe MRI for clinical diagnosis. During the past 30 years MRI has evolved as the standard workhorse in neurology and neurosurgery, while CAT scan has become the imaging technique of choice in an emergency (a standard CAT scan image of the head can be acquired in <5 min, but it takes more time to position a patient on the CAT scan table for scanning). MRI pictures are far superior in quality, resolution, and diagnostic accuracy. The major drawback of MRI is the length of time required for imaging, issues related to the high-power magnetic field, and claustrophobia (since the patient has to be positioned in the MRI magnet with the head at the center of the magnetic field). Also, a patient while being imaged cannot move, since any movement can distort the image quality. Because of these reasons many patients need sedation or even anesthesia (which is the primary subject of this chapter) during these imaging procedures, which may take anywhere from 30 min to as long as 90 min.
The next major innovation in imaging is the development of therapeutic techniques taking advantage of these high-quality MR images. MRI-guided biopsy of brain tumors is being carried out frequently. This is primarily applicable for brain tumors that are small and/or located deep inside the brain (regions like the thalamus and basal ganglia where surgical access is difficult and can result in significant neurological damage). MRI-guided biopsy causes minimal tissue injury. MRI-based imaging techniques have been developed, which can create reconstructed images of the brain based on which the exact trajectory for needle biopsy of a brain tumor can be visualized and carried out. This technique (called Brain Lab—a proprietary term) identifies the position of a patient’s head and creates MR images for viewing in the position patient is lying on the operating room (OR) table. This is a frameless stereotaxy imaging technique. MRI-guided laser thermocoagulation is another technique being developed for laser vaporization of brain tumors (as an alternative to standard craniotomy and tumor decompression). This technique permits laser vaporization of brain tumors through a small burr hole and the laser treatment can be visualized intermittently (based on the rise in temperature) and terminated when adequate treatment has been achieved in the lesion. This is the new area of therapeutic imaging part of MRI as against the diagnostic imaging during the beginning of MRI. Another specialized field that is fast coming up is the field of functional MRI (fMRI). fMRI can potentially image functional activity in the brain, and this permits visualization of neuronal activity, the exact part of the brain where specific functions like hearing, vision, touch, pain, memory, anger, thinking, and emotion are modulated. This has significant applications in tumor surgery, psychiatric disorders, developmental disorders in children, etc.
Principles of Nuclear Magnetic Resonance and Magnetic Resonance Imaging
Isidor Rabi discovered the nuclear magnetic resonance (NMR) property of nuclei. Subsequently, Felix Bloch and Edward Purcell independently described the MR property in bulk matter. Putting all this together, Paul Lauterbur and Raymond Damadian first generated images of living tissues with NMR in the 1970s. Subsequently, technology was refined further and with greatly improved techniques of image acquisition, storage, analysis, and interpretation culminated in the development of MRI capable of imaging the whole human body in the 1980s. The change in nomenclature from NMR to MRI was done to clarify that there is no radiation involved in MRI.
All atoms have a mass (due to the nucleus that has protons), electrons, and a magnetic property called spin. Thus each atom acts like a minute magnetic field, but due to the random alignment of the individual magnetic fields of various atoms the net magnetism of all atoms together is not significant. Human body has a high concentration of H + ions (because 60–70% of cell content is H 2 O). Hence when exposed to a high-power external magnetic field these H + ions (protons) align themselves parallel or antiparallel to the magnetic field (with a slight excess of protons being parallel to the magnetic field). This alignment of protons generates a macroscopic measurable magnetic field (called magnetic moment) ( Fig. 31.1 ). Each proton resonates (spins) at a specific frequency around its own magnetic field ( Fig. 31.2 ). This resonant frequency is proportional to the external magnetic field (42.57 MHz/T, called the Larmor constant). Gauss and Tesla are the units of measurement of magnetism (10,000 Gauss = 1 T). Our earth has a magnetic power of 2.3 G. In a 3 T magnetic field (this is the standard strength of human MRI), the resonant frequency of protons is 126 MHz. The interaction of the external magnetic field with the magnetic moment of the protons causes each nucleus to precess (alters the orientation of the spinning nucleus). Because of the abundance of H + ions in the human body (10 18 water molecules in an MRI voxel) proton signal from H + ions is captured and converted to an image in a standard conventional anatomical MRI. Voxel refers to the small volume of tissue from which signal’s are acquired in MRI. Similar to the “pixel” in a camera, the smaller the voxel, higher is the resolution of the images.


As per the quantum mechanics theory, energy level in the protons (also called spins) is a function of the alignment of the protons in the magnetic field. When protons are aligned parallel to the magnetic field (called B0 in MRI physics) the energy level is low. At this point protons are aligned and spinning along the z-axis (vertical). When the protons’ alignment is deflected away from the z-axis and along the x–y-axis the energy level of the proton increases ( Fig. 31.1 ). The amount of energy required to flip the protons away from the z-axis is very minimal—even the thermal energy at room temperature is adequate to flip the protons. All the signals that are acquired, stored, analyzed, and interpreted as an image in MRI are the small differences in energy state of the protons (spins) related to their alignment in the magnetic field. The net magnetization is the sum of the magnetization of all the protons in a given tissue, and the energy emanating from all the protons from the tissue in a voxel is the signal from the tissue. The vertical axis around which the spins rotate at Larmor frequency is the z-axis. At equilibrium more protons are aligned and spinning along the z-axis and few along the x–y-axis (horizontal axis).
Radio frequency (RF) magnetic field (resonance) : When an RF magnetic field is applied and ideally at a frequency equal to the Larmor frequency of the protons (H + ions), the alignment of the proton is altered from the z-axis toward the x–y-axis (horizontal axis) creating a high energy state in the protons. At this point there are more protons in the x–y-axis than along the z-axis (vertical axis) and hence more magnetization along the horizontal (x–y) axis. For the change in magnetization vector from vertical to horizontal axis two things have to happen: protons have to be moved to the high energy state and there should be equal number of protons (spins) in the high and low energy states. The RF magnetic field is referred to as B1. With RF pulse (B1) while the magnetization vector changes from vertical to horizontal axis the angle of tilt of the protons increases with time. By varying the amplitude and duration (typically in “milli” seconds) of RF pulse exposure any desired angle can be achieved. With a long enough RF signal exposure vertical magnetization vector will be zero and the vector will be rotated along the x–y plane. This angle of tilt of the protons is called the flip angle. In most cases there is a nonzero magnetization vector. It is the transverse component of the magnetization that generates the NMR signal. When B1 is turned off after a brief period, the magnetization of the protons will return to the z-axis along B0. In a standard MRI, the transverse magnetization can be measured with a receiver coil (which is the same as the RF coil). The amplitude of the RF signal is proportional to the concentration of protons (proton density in the tissue voxel being imaged): in the white matter 84% is water, while in the gray matter 71% is water. Basically the spins systematically give off the absorbed energy when its magnetization moves from the x–y axis to the z-axis. The amplitude of this RF signal, which is proportional to the transverse magnetization vector, will gradually decrease to zero. The molecular environment (chemical composition of the tissue) also determines the amplitude of the signal emanating from the protons.
Various Types of Signals Recorded
T1 relaxation time: T1 is the time required for the vertical magnetization to recover along the z-axis. This is the spin lattice relaxation time—energy given up to the surrounding molecules. T2 is the time required for the dissipation of transverse magnetization along the x–y axis. T2 relaxation is the spin–spin relaxation—this is the energy given up to the surrounding nuclei. The amplitude of T1 will depend on the composition of the tissue. Tissues with higher water content (like CSF) will have a higher T1 compared to tissues like fat that have a low water content. T2 is always less than T1 as magnetization is lost both to the surrounding spins as well as by dephasing. If the applied magnetic field is nonuniform spins in different parts of the tissue will rotate at different frequencies and they will quickly lose coherence (get dephased) and have much less transverse magnetization. This loss of transverse magnetization due to nonhomogeneous magnetic field is always less than T2 and is denoted by T2’. T2∗ is the combination of T2 and T2’. T2∗ is the signal measured in fMRI.
MRI signal: When a subject is placed in a high-power magnetic field (B0) all the protons will have roughly the same magnetic field in alignment and the signals also will be identical. Now if a second magnetic field is applied in a selected part of the sample being imaged (called the gradient coil) protons within the sample will emit signals of different frequency depending on their spatial location. Thus as the magnetic field varies across the subject, resonant frequency of the spins will also vary. The number of spins resonating at a particular frequency determines the amplitude of the signal in the spectrum of observed frequencies. From the gradient strength applied, direction of the signal and the frequency component the site of origin of the signal can be extrapolated on the sample. In whole body imaging the maximum linear field strength per voxel is 22 mT. Combining frequency gradient with a pulse of appropriate frequency and band width can excite a small slice, allowing slice-by-slice investigation of a selected region/organ in a subject. The small differences in microenvironment of tissues can be identified, detected, and imaged based on T1 and T2 relaxation times, proton density, and gradient in magnetic field. Presence of multiple MR properties permits greater flexibility in detection and imaging of tissue contrasts accurately with high spatial resolution. The tissue contrast depends on chemical composition of the tissue (structure), T1 and T2 relaxation times, and proton density. The combination of gradient wave forms and RF signal applied to generate images is called the pulse sequence.
MRI magnet consists of a large superconducting magnet since a high-power and homogeneous magnetic field is required. The superconducting electromagnet is kept cool with liquid helium. By cooling the magnet, electrical resistance is very low to negligible and there is no loss of energy. The circular flow of current generates a constant magnetic field oriented along the bore of the magnet. Additional coils are incorporated along the bore to keep the magnetic field strength homogeneous. Most cylindrical magnets used in clinical practice are of 1.5 T, and many 3-T magnets also have been introduced in the past 10 years. Four more additional coils are incorporated of which three of them act as gradient coils (to create small perturbations in magnetic field in the orthogonal planes). Since the precision frequency of the protons is related to the magnetic field strength, these gradient coils encode the spatial location of the protons within the body based on the precision frequency. An RF coil gives the burst of RF energy required to perturb/alter the magnetization of the spins within the body. Most MRI systems have a whole body RF coil permanently installed next to the gradient coil. This gives a homogeneous excitation of the large volume of tissue within the bore of the magnet. Some RF coils are designed to receive the echo signals from the protons. However, in most systems separate small coils are incorporated close to the region being imaged.
Intraoperative MRI : Intraoperative MRI is considered the third biggest advancement in neurosurgery after bipolar cautery and the operating microscope. In modern neurosurgery the importance of precisely targeting the region of interest cannot be overemphasized. In a brain tumor successful resection depends on the surgeons’ ability to delineate the margins of the tumor. In seizure surgery the epileptic foci to be resected has to be registered with the functional images and the localization has to be of submillimetric precision. Brain tumors like glioma do not have a well-defined capsule, and in the surgical field, identifying the line of separation between the tumor and the normal brain can be challenging even for a trained eye. Neuronavigation tools (also called Brain lab) have been developed to facilitate localization of the lesion in the operating field. In comparison to conventional stereotaxy systems (which have been in use for over 50 years now) MRI-based navigation systems have been a significant improvement in imaging technology. MRI-based navigation systems combine the advantages of a stereotaxic frame with the precision of a high-resolution MRI. Both frame-based and frameless MRI-based neuronavigation systems use a 3D preoperative MR image of the brain, and this image is merged with the patients’ surface anatomy during the registration process. Frame-based systems are accurate and precise in localizing the target (within 1–2 mm); however, the presence of a stereotaxy frame limits the surgical field. Only procedures done through a burr hole (deep brain stimulator implantation, brain biopsy, etc.) are possible with conventional frame-based stereotaxy.
The modern MRI-based neuronavigation systems (like Brain Lab, Stealth system) being frameless systems they permit access for any type of craniotomy without limiting the surgical field. In the frameless navigation system using an electromagnetic or optical sensor the movement of the surgical instruments in the operating field can be tracked accurately. The navigation system can project the position of the surgical instrument in the brain relative to the lesion. The frameless MRI-guided imaging system gives a reconstructed image of the brain in the operating field. The reconstructed image of the brain with the lesion to be operated upon (based on the MR image acquired before surgery) can be seen in all three planes—sagittal, axial, and coronal. However, there are some drawbacks in the frameless navigation system. Being a reconstructed image (and not a real-time image) once the cranium is open the brain shifts and the neuroanatomy is altered because of CSF drainage, brain retraction, resection of tumor, etc. Hence reconstructed image is not reflective of the real-time anatomy, as would be expected during surgery. Brain shift of more than 10 mm has been documented within 1 h of opening of the duramater and before the excision of the lesion. This error can be exaggerated in the presence of a preexisting loss of brain parenchyma or hydrocephalos. However, if an MR image is acquired during the surgery [intraoperative MRI (iMRI)], this new MR image can be used for neuronavigation and this image would be as close to the real-time anatomy as it can be. This intraoperative image can identify the extent of resection of the lesion, any distortion in neuroanatomy induced by surgery, presence of blood clot, etc. These are some of the factors that lead to the development of iMRI in the 1990s.
Over the past 20 years many types of iMRI have been designed. The earliest version was a 0.5-T magnet of a double donut open configuration designed at the Brigham and Women’s Hospital in Boston. There was a 70-cm space between the magnets, which was available for the surgeon to operate. While this design permitted MRI during surgery, it introduced a whole set of issues like (1) all surgical instruments, electrocautery, drill, suction instrument, etc., were required to be made of nonferrous MRI-safe material; (2) access to the surgical site was limited; and (3) the entire OR had to be redesigned with shielding of the wall, flooring, and roof. The next model of iMRI was a portable magnet, which could be stored in a protected place (Faraday’s cage) and brought out for imaging when required. Initial models were 0.12–0.15 T and subsequently a 0.5-T portable MRI was introduced. With a portable magnet, redesigning of the entire OR was not required and surgical instruments could be moved away during imaging (hence special nonferrous instruments were not required). However, since portability limits the size and the strength of the magnet in terms of Tesla the image resolution was not the best in this model. The next generation of iMRI was a closed bore magnet. This magnet is designed to be stored in a garage when not in use. The magnet is fixed on rails and is brought into the OR when imaging is required. This model of iMRI has a higher strength magnet (3 T) and gives a good image resolution. The protocol for iMRI followed by most neurosurgeons is intermittent imaging during surgery. When imaging is required the operating field is draped, all the surgical instruments and surgical devices are moved outside the 5-G line, and the magnet is brought out for imaging. Thus nonferrous surgical instruments and devices are not required with this model. The OR has a clearly marked demarcation of the 50-G and 5-G lines. This configuration of iMRI permits installation of one magnet between two ORs. MRI-guided laser thermocoagulation of brain tumors is also being carried out in some centers. A robotic arm is also being developed for manipulating the operating area during imaging.
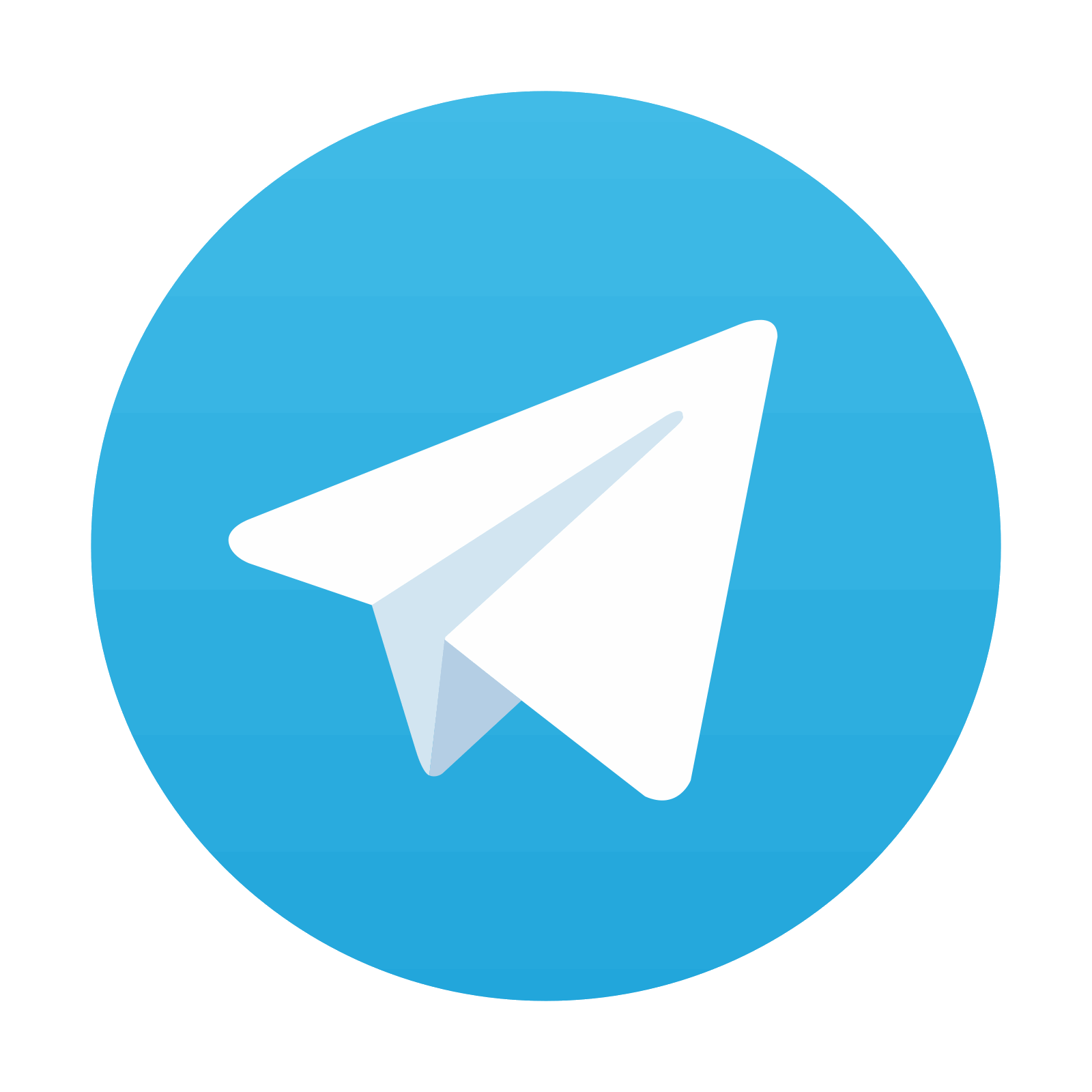
Stay updated, free articles. Join our Telegram channel
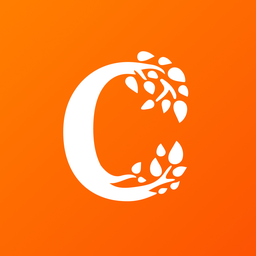
Full access? Get Clinical Tree
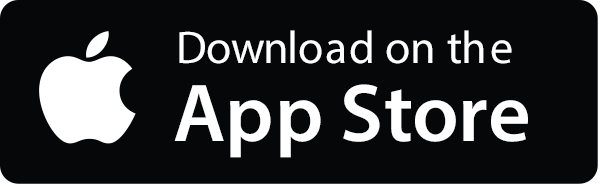
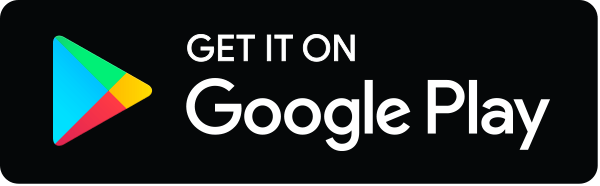