Introduction
In 1695, from well water in Epsom, England, Dr. Nehemiah Grew prepared Epsom salts, a name still given to magnesium sulfate. The biological significance of magnesium as a constituent of plants has been known since the 18th century, with magnesium ion an essential component of chlorophyll.
Hypomagnesemia is observed in about 10% of hospitalized patients and especially critically ill patients who often have other coexisting electrolyte abnormalities and long hospitalizations. Hypomagnesemia is associated with numerous complications including ventricular arrhythmia, coronary artery spasm, prolonged hospitalization, and increased mortality ( ) .
While there are no practical methods to measure overall magnesium status, the measurement of total magnesium remains the usual test for assessing clinical magnesium status. Ionized magnesium methods are available, with reliability not yet to the standard of ionized calcium methods. The Mg ion sensors for these electrodes were developed from studies on over 200 ionophores produced by the late Dr. Wilhelm Simon and his colleagues.
Magnesium distribution and regulation in the blood
The human body contains ∼1 mol (24 g) of magnesium, with ∼53% in the skeleton and ∼46% in soft tissues such as skeletal muscle, liver, and myocardium. Magnesium is primarily an intracellular ion, with only ∼1% in blood and ECFs ( ) . Like calcium, magnesium in serum exists as protein-bound (24%), complex-bound (10%), and ionized (66%) forms ( ) . As with calcium, the pH of blood apparently affects the binding of Mg ions by proteins in the blood ( ) .
The recommended dietary intake of magnesium is 10–15 mmol/d. Rich sources of magnesium include green vegetables, meat, grains, and seafood. The small intestine absorbs from 20% to 60% of the dietary magnesium by both active and passive transport mechanisms, depending on the need. Secretions from the upper GI tract and especially the lower GI tract contain magnesium. This explains why prolonged diarrhea, vomiting, inflammatory bowel disease, surgical removal of intestinal segments, and pancreatitis often cause magnesium depletion.
Mg homeostasis mainly involves the kidneys, the small intestine, and bones, with GI absorption and renal excretion the most important mechanisms. Active transcellular Mg uptake is through specific Transient Receptor Potential Melastatin 6 and 7 (TRPM6 and TRPM7) Mg ion channels in the small intestines, with paracellular (between cells) absorption driven by electrochemical gradients developed by ion movements such as calcium through epithelia in the small intestines ( ) .
The kidneys are the primary site of Mg homeostasis and play a key role in regulating and maintaining Mg balance. The proximal tubule reabsorbs 15%–20% of filtered Mg, the distal tubule 5%–10%, and almost none is reabsorbed in the collecting ducts ( Fig. 6.1 ). The thick ascending limb (TAL) of the loop is the major site, reabsorbing about 60%–70% of filtered Mg ( , ) , promoted by several cotransporters and ion channel facilitators. These include the proteins claudin-16 and claudin-19, which promote Mg ion reabsorption in the TAL. Claudins are membrane proteins in the “tight-junctions” between cells that appropriately facilitate or block the flow of molecules and ions through the paracellular (between-cell) spaces. In the TAL, claudins reabsorb mainly Mg ions and a small amount of Ca ions. Claudins are closely regulated by the calcium-sensing receptors (CaSR) that inhibit their ability to reabsorb Mg ions. The actions of these transporters are shown in Fig. 6.1 .

Magnesium absorption is fine-tuned in the distal convoluted tubule (DCT) that reabsorbs 5%–10% of filtered Mg via the Mg ion channel TRPM6. TRPM6 are protein cation channels embedded in the cells of the DCT that allow Mg ions to flow into cells. When the body needs additional Mg, the TRPM6 channels allow Mg ions to be reabsorbed from the DCT fluids into the blood. When the body has sufficient or excess Mg, the TRPM6 channels allow Mg ions to be lost from the DCT cells into the urine, and the CaSRs are activated to inhibit Mg ion absorption ( , ) .
The renal threshold for magnesium is ∼0.60–0.85 mmol/L (∼1.46–2.07 mg/dL). Because this is close to the normal serum concentration, the kidneys rapidly excrete even slight excesses of magnesium in the blood. Factors that affect tubular reabsorption of magnesium in the kidney are listed in Table 6.1 .
Factors that increase Mg absorption | Factors that decrease Mg absorption |
---|---|
PTH | Hypermagnesemia |
Insulin | Hypercalcemia |
Calcitonin | Diuretics |
Glucagon | Aminoglycoside antibiotics |
ADH | Amphotericin B (antifungal) |
Aldosterone | Cisplatin (chemotherapeutic) |
Metabolic alkalosis | Cyclosporin (immunosuppressant) |
Dietary Mg deficiency | Mutations to genes that code for Mg ion cotransporters and ion channels (claudins, TRPM6) |
Under normal physiologic conditions, both passive and active transport systems absorb magnesium in the GI tract, with the duodenum absorbing 11%, the jejunum 22%, the ileum 56%, and the colon 11% ( ) . A transcellular transport mechanism relies on active transporters TRPM6 and TRPM7. These transporters strip away the hydration shell of magnesium allowing it to pass through the channels. Passive paracellular diffusion occurs in the small intestine and is responsible for 80%–90% of overall magnesium absorption. A high Mg concentration of 1–5 mmol/L in the lumen drives this passive transport, which relies on electrochemical and solvent diffusion to pass Mg ions through the tight junctions between intestinal cells ( ) .
Although parathyroid hormone (PTH) has a role in regulating both magnesium and calcium, no specific mechanism for regulating Mg ion homeostasis in the blood has yet been described. Similar to its effect on calcium, PTH increases renal reabsorption of magnesium and enhances absorption of magnesium in the intestine. Paradoxically, both hypermagnesemia and hypomagnesemia can depress the secretion of PTH. Hypermagnesemia acts similarly to hypercalcemia by binding to CaSRs on the parathyroid gland and shutting off PTH production. Hypomagnesemia apparently blocks ion channels and suppresses the release of PTH, which leads to hypocalcemia. Magnesium regulation may depend on a complex interdependence of renal excretion, GI absorption, and bone exchange, and the ionized intracellular Mg concentration may be the regulatory signal ( ) . In addition to PTH, insulin, aldosterone and vitamin D may play a role in magnesium regulation ( , ) .
The parathyroid gland is far more sensitive to a decrease in ionized calcium than magnesium ( , ) . As shown in Fig. 6.2 , a 0.065 mmol/L decrease in blood ionized calcium (about 5%) increased the PTH concentration about fourfold, while a 0.03 mmol/L decrease in ultrafiltrable magnesium (also about 5%) produced no detectable PTH response, as shown in Fig. 6.3 .


Regulation of magnesium is not as well characterized as that of calcium, with a number of hormones possibly having an effect on renal magnesium reabsorption. Hormones that have some effect on magnesium transport in the TAL include PTH, calcitonin, glucagon, ADH, and β-adrenergic agonists, all of which are coupled to adenylate cyclase in the TAL ( ) . While the effects of these hormones may be related to an increase in luminal positive voltage and an increase in paracellular permeability, their importance in magnesium homeostasis is not known.
PTH increases the release of magnesium from bone, increases the renal reabsorption of magnesium, and, with vitamin D, enhances the absorption of magnesium in the intestine. As noted earlier, chronic or severe acute hypomagnesemia can inhibit secretion of PTH, a mechanism by which hypomagnesemia causes hypocalcemia. Aldosterone apparently inhibits the renal reabsorption of magnesium, an effect opposite that of PTH.
Insulin has a hypermagnesemia effect by increasing both intestinal and renal absorption of magnesium. Insulin has its hypermagnesemia action in the kidney as a factor that binds to receptors that activate the TRPM6 ion channels and ion transporters ( ) .
Physiology
Magnesium is an essential cofactor in well over 300 enzymes in systems involved in almost every aspect of biochemical metabolism, such as DNA and protein synthesis, glycolysis, and oxidative phosphorylation ( ) . Adenylate cyclase and sodium–potassium–adenosine triphosphatase (Na–K ATPase) are vital enzymes that require Mg ions for proper function. Mg also supports immune functions ( ) , and Mg concentrations correlate with the levels of several immune mediators such as interleukin-1, tumor necrosis factor-α, interferon-γ, and the neurotransmitter peptide substance P. Moreover, Mg ions help to stabilize cell membranes and ion channels, protein and nucleic acid synthesis, regulate cardiac and smooth muscle tone, control mitochondrial functions, and support bone cell integrity ( ) .
Magnesium ion acts as a calcium-channel blocker by affecting the influx of Ca ions at specific sites in the vascular membrane. In a healthy arterial cell with an adequate supply of magnesium, the gates are stabilized and control the entry of Ca ions. Magnesium deficiency promotes the accumulation of intracellular Ca and Na ions, leading to a state of greater contractility ( ) .
Other conditions associated with hypomagnesemia include the following:
- •
The effects on myocardial function and blood pressure ( ) .
- •
The implications of magnesium depletion during open-heart surgery ( ) and critical care ( ) .
- •
A possible role in migraine headaches, asthma, and chronic fatigue syndrome ( ) .
Hypomagnesemia
Causes and clinical conditions associated with hypomagnesemia
Hypomagnesemia, defined as a serum magnesium of <0.75 mmol/L (1.8 mg/dL) ( ) , with the common causes of hypomagnesemia shown in Table 6.2 .
|
|
|
|
|
|
|
|
|
Critical illness
Magnesium deficiency is found in a large percentage of critically ill patients ( ) , and the presence of hypomagnesemia on admission is associated with an increased mortality rate ( ) . The causes of hypomagnesemia in critically ill patients are mainly from gastrointestinal disorders or renal loss of Mg. GI disorders such as prolonged diarrhea, vomiting, inflammatory bowel disease, surgical removal of intestinal segments, and pancreatitis are associated with magnesium depletion ( ) . Many of the causes of Mg deficiency in ICU patients are among those listed in Table 6.2 . Renal loss may be enhanced by drugs and alcoholism ( , ) . Although magnesium deficiency is more common in critically ill patients, hypermagnesemia is more often associated with a poor outcome than is hypomagnesemia ( ) , most likely because hypermagnesemia is almost always a secondary effect of kidney disease that causes higher mortality.
In pediatric patients, magnesium was the most common electrolyte abnormality found in pediatric intensive care unit patients ( ) , and ionized magnesium may be decreased in many critically ill pediatric patients who have normal total magnesium concentrations ( ) . In this study, the use of albumin-corrected total magnesium was not reliable in estimating the ionized magnesium.
Some studies conclude that either total or ionized magnesium measurements may be used to follow magnesium status in patients in critical care ( , ) , while others concluded that the ionized magnesium was more specific ( , ) . Another study concluded that red blood cell magnesium was the best parameter to measure because it gave a higher incidence of hypomagnesemia (37%) than did either ionized magnesium (22%) or total magnesium (16%) ( ) . See the section “Ionized Mg versus total Mg” at the end of this chapter.
In patients during abdominal surgery who were not given massive transfusions, changes in ionized and total serum Mg concentrations correlated closely ( ) , and total serum Mg adequately screened for hypomagnesemia. Magnesium supplementation during cardiopulmonary bypass appeared to significantly benefit patients with hypomagnesemia by preventing ventricular tachycardia ( , ) .
In a review of 253 patients admitted to the ED, mild, moderate, and severe hypomagnesemia were found in 19.5%, 9.1%, and 2.5% of the patients, although these levels did not relate to mortality ( ) . These were related to drugs, alcoholism, kidney failure, and poor diet.
Cardiac disorders
In cardiovascular disease, myocardial hypoxia accompanied by cellular magnesium deficit will more rapidly deplete ATP, leading to disruption of mitochondrial function and structure. With Mg ion important in energy metabolism, ion movements via Na–K-ATPase, calcium channel regulation, myocardial contraction, and other cardiac functions, the heart is particularly vulnerable to magnesium deficiency. Hypomagnesemia can disrupt mitochondrial function and production of ATP, which promote loss of myocardial potassium. These conditions can contribute to coronary vasospasm, arrhythmias, atrial fibrillation, infarction, and sudden death ( ) . A low magnesium concentration enhances the potency of vasoconstrictive agents that can produce sustained constriction of arterioles and venules. Furthermore, a low serum magnesium promotes endothelial cell dysfunction that inhibits nitric oxide (NO) release and promotes a proinflammatory, prothrombotic, and proatherogenic environment ( , ) .
Table 6.3 summarizes the cardiac disorders known to be associated with loss of myocardial and/or serum magnesium ( , ) .
|
|
|
|
|
|
|
|
|
Magnesium affects vascular tone by modulating the vasoconstrictive effects of hormones such as norepinephrine and angiotensin II: A high Mg:Ca concentration ratio antagonizes their effects, whereas a low Mg:Ca ratio enhances their activity ( ) . Ca and Mg ions compete for binding to the contractile proteins, with Ca ions initiating contraction (vasoconstriction) and Mg ions inhibiting contraction (vasodilation).
Postoperative hypomagnesemia is common following cardiac operations and is often associated with atrial fibrillation and arrhythmias ( ) . Hypomagnesemia also impairs the release of NO from coronary endothelium, which promotes vasoconstriction and coronary thrombosis in the early postoperative period ( ) . Intravenous magnesium sulfate solution has been shown to reduce the incidence of postoperative atrial fibrillation ( ) . However, overdosing magnesium must be avoided ( , ) . In patients undergoing surgery with cardiopulmonary bypass, plasma ionized (but not total) magnesium was decreased significantly by 24 h after bypass ( ) . By correcting serum magnesium levels during cardiopulmonary bypass, the incidence of ventricular tachycardia was reduced from 30% to 7% ( ) .
The benefit of administering magnesium for myocardial infarction is controversial. Magnesium therapy reduced the frequency of ventricular arrhythmias in acute myocardial infarctions (AMI) ( ) . The LIMIT-2 Study on over 2000 patients found a distinct benefit of administering Mg early to patients with suspected AMI ( ) , and a rationale for the benefit of magnesium was presented ( ) . A key factor was thought to be the timing of magnesium supplementation, which might be required to achieve benefits in high-risk patients, before reperfusion occurs. However, the very large MAGIC trial did not find a benefit of early administration of magnesium ( ) . A more recent review was inconclusive about magnesium being routinely administered for AMI ( ) .
In a study of pediatric patients undergoing surgery for congenital heart defects, magnesium supplementation was so effective in preventing ectopic tachycardia (none in 13 patients) compared to the placebo group (4 in 15), that the study was terminated after 28 patients ( ) .
Drugs
Several drugs, including diuretics, gentamicin and other aminoglycoside antibiotics, cisplatin, and cyclosporine increase the renal loss of magnesium and frequently result in hypomagnesemia. Loop diuretics (furosemide, and others) significantly increase magnesium excretion by inhibiting the electrical gradient necessary for magnesium reabsorption in the TAL. Long-term thiazide diuretic therapy also may cause magnesium deficiency, through enhanced magnesium excretion by reducing levels of the TRPM6 ion channels in the TAL cell membranes ( , , ) .
Many nephrotoxic drugs, including cisplatin, amphotericin B, cyclosporine, and tacrolimus, can cause profound hypomagnesemia by increasing urinary magnesium wasting, possibly involving a variety of mechanisms that inhibit the TRPM6 channels. Good urine flow should be maintained during therapy with these drugs to prevent kidney toxicity. The effects of these and other drugs that cause hypomagnesemia are presented in a review ( ) . Urinary magnesium wasting caused by the administration of calcineurin inhibitors such as cyclosporine and tacrolimus is partly the reason that hypomagnesemia frequently develops after kidney transplantation. Other causal factors in these patients include posttransplantation volume expansion, metabolic acidosis, and insulin resistance ( ) .
Aminoglycosides such as gentamicin inhibit reabsorption of magnesium in the renal tubule. Because hypomagnesemia intensifies the toxic side effects of these drugs, hypomagnesemia should be avoided. Aminoglycosides are thought to produce magnesium wasting by inducing the activity of the CaSRs on the ascending limb and distal tubule of the kidney ( ) .
Proton pump inhibitors (PPI) such as omeprazole (Prilosec) and lansoprazole (Prevacid) are often used to treat ulcers and gastroesophageal reflux disease (GERD) by reducing stomach acid production. However, with long-term use, they can cause hypomagnesemia by inhibiting the Mg ion transporters TRPM6 and TRPM7 in the intestines ( , ) .
Diabetes
Hypomagnesemia is common in patients with diabetes ( , ) , with a serum Mg <0.7 mmol/L strongly associated with type 2 diabetes. Patients with type 2 diabetes and hypomagnesemia show a more rapid disease progression and development of insulin resistance. Consequently, patients with type 2 diabetes and hypomagnesemia enter a vicious circle in which hypomagnesemia causes insulin resistance and insulin resistance causes hypomagnesemia ( ) . Hypomagnesemia may also intensify complications frequently associated with diabetes, such as retinopathy, hypertension, cardiovascular disease, and increased platelet activity and thrombosis. In the pediatric population, hypomagnesemia was a strong, independent risk factor for insulin resistance in obese children ( ) . Dietary Mg supplementation for many patients with type 2 diabetes improved glucose metabolism and insulin sensitivity ( ) .
In a study of 128 patients with chronic renal failure, those with diabetes had both total and ionized Mg about 10% lower than in the nondiabetic renal patients ( ) , and another report concluded that a low serum Mg was associated with a more rapid decline of renal function in patients with type 2 diabetes ( ) .
The mechanism of magnesium deficiency in diabetes appears to be abnormal intracellular–extracellular distributions of Mg ions caused by insulin and other factors. Intracellular Mg ions regulate glucokinase, potassium-ATP (KATP) channels, and L-type (long-lasting voltage-dependent) Ca ion channels in the pancreatic β-cells, which activate insulin secretion. Such mechanisms make intracellular Mg ions a direct factor in the development of insulin resistance.
Insulin helps regulate Mg ion homeostasis in the kidney, where it activates both renal Mg ion channels and transporters (such as TRPM6) that determine the final urinary Mg excretion in the DCT ( ) . Magnesium deficiency may also be related to increased urinary loss of magnesium from osmotic diuresis caused either by glycosuria or hormonal imbalances, such as decreased PTH and altered vitamin D metabolism.
In 1992, the American Diabetes Association issued a statement about magnesium and diabetes ( ) . At that time, the ADA stated that there was a strong relationship between hypomagnesemia and insulin resistance and that magnesium supplements should be given to all patients with documented hypomagnesemia and symptoms, but did not recommend magnesium supplementation to all patients with diabetes. In 2013, the ADA issued a statement emphasizing the importance of a balanced diet to promote healthful eating patterns, emphasizing a variety of nutrient-dense foods in appropriate portion sizes, to improve overall health and specifically to attain individualized glycemic, blood pressure, and lipid goals. Their brief statement about magnesium supplementation was weakly put: “Evidence from clinical studies evaluating magnesium … supplementation to improve glycemic control in people with diabetes is conflicting” ( ) .
Dietary deficiency
Dietary surveys show that almost half of people in the United States consume less than recommended amounts of magnesium, with older men and adolescent males and females most likely to have low intakes. Green vegetables such as spinach contain magnesium in the chlorophyll of these plants. Beans and peas, nuts and seeds, and whole, unrefined grains are also good sources of magnesium ( ) . Adequate dietary Mg is continually necessary because the GI tract cannot increase Mg absorption during dietary Mg shortage.
Alcoholism
Chronic alcoholism has long been associated with hypomagnesemia ( , ) . Both acute and chronic alcohol consumption increase renal magnesium excretion. Hypomagnesemia in alcoholic patients apparently results from alcohol-induced tubular dysfunction along with a combination of dietary magnesium deficiency, vomiting, and diarrhea. Infusion of glucose solutions induces insulin secretion, which shifts magnesium back into cells. Both total and ionized magnesium apparently increase toward normal after 3 week of abstinence from alcohol ( ) .
Cellular hypoxia
Cellular hypoxia leads to depletion of ATP, which causes cellular loss of Mg and K ions. Chronic hypoxic conditions, such as decreased cardiac output, can also result in hypomagnesemia.
Preeclampsia of pregnancy
Preeclampsia is a hypertensive disorder that occurs in ∼5% of pregnancies and causes significant morbidity and mortality. The pathogenesis of preeclampsia is complex, but it begins with abnormal placental implantation and development that leads to endothelial and immunologic dysfunction. Clinical features include the following: hypertension, proteinuria, and dysfunction of the kidneys, heart, lungs, and CNS. Also, fetal growth may be affected.
Because magnesium requirements increase during pregnancy, hypomagnesemia is common in preeclampsia and eclampsia (development of seizures). Treatment with magnesium salts is a long-accepted practice for these conditions, although extreme hypermagnesemia sometimes results from excessive doses ( ) . The fetus may also be affected by hypermagnesemia in such cases.
Diarrhea
As noted in the section “Critical Illness,” secretions from the lower GI tract are relatively rich in Mg so that diarrhea, malabsorption syndromes, bowel resection, etc. are common causes of Mg depletion.
Burns
Loss of skin integrity via burns is associated with a general loss of fluid and electrolytes.
PTH deficiency
Because PTH increases tubular reabsorption of Mg in the kidney, a deficit of PTH, such as in primary hypoparathyroidism, will lead to hypomagnesemia. Sepsis inhibits PTH secretion and is also associated with hypomagnesemia ( ) .
Other diseases
Magnesium deficiency may promote the development and progression of renal stone formation and other renal calcification ( ) . Magnesium ions may prevent calcification by chelating anions that would otherwise form insoluble salts with calcium.
Biochemical manifestations of hypomagnesemia
Hypokalemia . Hypokalemia is common in patients with Mg deficiency and vice versa. Indeed, patients with Mg depletion have a renal loss of potassium caused by an increased potassium secretion in the connecting tubule and the cortical collecting tubule. In the kidneys, K + is absorbed across the basolateral membrane via Na–K ATPase and secreted into the lumen of the connecting tubule and cortical collecting tubule. This process is mediated by renal outer-membrane potassium channels (ROMK), which require ATP. At normal intracellular Mg ion concentrations, these channels move more K ions into cells. With a lack of intracellular Mg ions, K ions move freely through the ROMK channels, increasing cellular potassium efflux that may be detected as increased potassium in the urine ( ) .
Hypocalcemia . Mg deficiency is often associated with hypocalcemia ( ) . Patients with combined hypocalcemia and hypomagnesemia often have low levels of PTH. This is caused by Mg deficiency inhibiting the release of PTH from the parathyroid gland. Because parenteral Mg stimulates PTH secretion, reduced PTH secretion contributes to hypocalcemia in Mg deficiency ( ) .
Evaluation of magnesium status in patients
Magnesium should be measured during the initial examination of all patients with poor food intake, malabsorption disorders, hypokalemia, or hypocalcemia, as well as those taking magnesium-depleting agents, such as diuretics, alcohol, or aminoglycosides. A patient with hypomagnesemia may show a variety of nonspecific symptoms, such as weakness, muscle cramping, and rapid heartbeat ( ) . While measurements of total magnesium concentrations in serum remain the usual diagnostic test for detection of magnesium abnormalities, it has limitations:
- •
25%–30% of magnesium is protein-bound. Therefore, as with total and ionized calcium, total magnesium may not reflect the physiologically active free ionized magnesium. This is discussed later in the section “Ionized magnesium versus total magnesium.”
- •
Because Mg is primarily an intracellular ion, serum concentrations will not necessarily reflect the intracellular status of magnesium ( ) . Even when tissue and cellular magnesium is depleted by as much as 20%, serum magnesium concentrations may remain normal.
The magnesium load test may be the definitive method for detecting body depletion of magnesium, as was shown in 13 patients with pancreatitis and a normal concentration of magnesium in serum ( ) . However, because a magnesium load test requires >48 h to complete, its clinical use is limited. After collection of a baseline 24-h urine, 30 mmol (729 mg) of magnesium in a 5% dextrose solution is administered intravenously (i.v.) as another 24-h urine is collected. Individuals with adequate body stores of magnesium will excrete 60%–80% of the magnesium load within 24 h, while magnesium-deficient patients will excrete <50% ( ) .
Urinary magnesium may help confirm the cause of a magnesium deficit. If it is >25–30 mg/d, renal loss is suspected; if <20 mg/d, nonrenal causes should be suspected, such as inadequate intake or GI loss.
In addition to renal loss, acute hypomagnesemia can result from intracellular shifts of magnesium after the administration of glucose or amino acids ( ) . This effect is pronounced after starvation or insulin treatment for hyperglycemia.
Treatment of hypomagnesemia
For patients at risk for magnesium depletion, magnesium supplements may be given to prevent hypomagnesemia. Magnesium replacement therapy may be warranted if serum Mg is <0.5 mmol/L (<1.2 mg/dL) ( ). However, because serum Mg does not necessarily reflects the total body Mg status, patients at risk for magnesium deficiency or with symptoms consistent with hypomagnesaemia should be considered for treatment even with normal serum Mg concentrations ( ) . In acute-care patients, hypomagnesemia is often associated with coronary artery disease and coronary bypass surgery, malignancy, chronic obstructive pulmonary disease, and alcoholism. Among chronic diseases, alcoholism, liver disease, and carcinoma were commonly associated with hypomagnesemia ( ) .
If magnesium infusion is necessary, as a guide, 25 mmol of MgSO 4 solution given intravenously over 12 h increased serum magnesium concentration to about 1.5 mmol/L, while 50 mmol per 12 h increased serum magnesium to about 2 mmol/L ( ) . Renal function should be assessed to guide supplementation and prevent hypermagnesemia ( ) . In another study, administration of 1 g of MgSO 4 in solution to critically ill patients increased total Mg by 0.11 mmol/L and ionized Mg by 0.05 mmol/L ( ) .
In general, mild hypomagnesemia with no or only mild symptoms can be treated with an oral supplement, whereas parenteral Mg supplementation is indicated if Mg concentration is <0.5 mmol/L or if the patient presents with significant symptoms ( ) . For critically ill patients with mild-to-moderate hypomagnesemia, empirically administering 1 g (4 mmol) of IV Mg will increase the serum Mg concentration by 0.08 mmol/L within 18–30 h. Trials of patients with acute myocardial infarction suggest an initial bolus (e.g., 2 g (8 mmol)) followed by continuous infusions of up to 16 g (65 mmol) over 24 h. Guidelines for treating severe hypomagnesemia are that lower doses (<6 g MgSO 4 ) can be given over a period of 8–12 h, whereas higher doses may be administrated over a period of 25 h or longer ( ) . These timings are made especially important due to the combined slow distribution of Mg in tissues and the rapid renal excretion of magnesium ( ) .
When drugs that deplete magnesium, such as gentamicin, diuretics, cisplatin and cyclosporine are given, MgSO 4 solution can be given intravenously once or more daily, as guided by serum magnesium measurements. Women with toxemia of pregnancy (eclampsia) are sometimes given very large doses of magnesium sulfate intravenously (175 mmol/24 h). This should be monitored with measurements of serum magnesium, because potentially dangerous hypermagnesemia to concentrations of 4.0 mmol/L and above can occur ( ) .
Hypermagnesemia
Causes of hypermagnesemia
Significant hypermagnesemia is almost always associated with impaired kidney function, because healthy kidneys can readily excrete excess magnesium loads. A well-functioning kidney handles a magnesium load by diminishing Mg absorption in the thick ascending limb of the loop, where over 50% of filtered magnesium is normally reabsorbed ( ) . While mild elevations in plasma magnesium may be seen in 10%–15% of hospitalized patients, such patients usually have some degree of impaired kidney function. More severe hypermagnesemia can occur when excessive doses of magnesium-containing antacids, enemas, or parenteral nutrition are administered to patients with renal insufficiency ( ) . Hypermagnesemia is sometimes seen in adrenal insufficiency, probably related to diminished aldosterone causing volume depletion and hemoconcentration. The most common cause of dramatically increased plasma magnesium without chronic renal failure is found in treatment for eclampsia of pregnancy, for which large amounts of magnesium salts are given.
Kidney impairment . As kidney function declines, plasma magnesium levels rise because the only regulatory system that is able to remove magnesium is urinary excretion ( , ) . In patients with end-stage kidney disease or on dialysis, magnesium intake must be carefully monitored to maintain control of the plasma magnesium concentration. For example, hypermagnesemia (defined as a serum magnesium greater than 1.5 mmol/L) can occur with magnesium intake as low as 280 mg/day, which is within the range of average intake by the general population of about 200–340 mg/day, depending on weight. Because symptomatic hypermagnesemia can be caused by magnesium given as antacids or laxatives in typical therapeutic doses ( ) , these agents should be avoided in patients with renal impairment.
Magnesium infusion . In pregnant women with severe preeclampsia or eclampsia, parenteral magnesium is commonly given intravenously to decrease neuromuscular excitability ( ) . The usual plasma concentration achieved is 6–8.4 mg/dL or 2.5–3.5 mmol/L, but much higher levels can occur. Frequent complications of hypermagnesemia are hypocalcemia and hyperkalemia, as hypermagnesemia can suppress the release of PTH ( ) . Neonates with hypermagnesemia may also have hyperkalemia and hypocalcemia, along with hypotonia, all of which increase the need for neonatal intensive care ( ) .
Oral ingestion . Ingestion of very large oral doses of magnesium may exceed renal excretory capacity, especially if either chronic kidney disease or acute kidney injury is present ( ) . Accidental overdose can also occur with over-the-counter products, such as Epsom salts (MgSO 4 ) or laxatives ( ) .
Enemas . Enemas usually contain high concentrations of MgSO 4 , so that substantial quantities of magnesium can be absorbed from the large bowel following an enema. Even in normal subjects, enemas containing 400–800 mmol of MgSO 4 can raise the plasma magnesium concentration to 7.2–19.2 mg/dL (3–8 mmol/L). This amount of magnesium given as an enema to patients with kidney failure can be fatal ( ) .
Symptoms of hypermagnesemia
Most symptoms of hypermagnesemia are from neuromuscular effects, cardiovascular effects, and hypocalcemic effects. Symptomatic hypermagnesemia may occur with the following plasma concentrations of magnesium ( ) :
- •
2–3 mmol/L (4.8–7.2 mg/dL): Nausea, flushing, headache, lethargy, drowsiness, and diminished deep tendon reflexes.
- •
3–5 mmol/L (7.2–12 mg/dL): Somnolence, hypocalcemia, absent deep tendon reflexes, hypotension, bradycardia, and electrocardiogram (ECG) changes.
- •
Above 5 mmol/L (12 mg/dL): Muscle paralysis leading to quadriplegia, apnea, and respiratory failure that usually precedes complete heart block and cardiac arrest.
Neuromuscular effects are the most common complication of hypermagnesemia, because increased magnesium decreases impulse transmission across the neuromuscular junction, and this can be detected by diminished deep tendon reflexes, which are usually noted when the plasma magnesium concentration reaches 2–3 mmol/L (4.8–7.2 mg/dL) ( ) .
Cardiovascular effects are related to excess intracellular Mg blocking cardiac K channels. Hypotension, conduction defects, and bradycardia begin to appear at a plasma magnesium concentration of 2–2.5 mmol/L (4.8–6 mg/dL). ECG changes may occur at concentrations of 2.5–5 mmol/L (6–12 mg/dL). Complete heart block and cardiac arrest may occur at a plasma magnesium concentration above 7.5 mmol/L (18 mg/dL).
Moderate hypermagnesemia can inhibit the secretion of PTH, leading to a decreased plasma calcium concentration. The fall in the plasma calcium concentration is usually transient and asymptomatic, although ECG abnormalities may occasionally occur.
Treatment of hypermagnesemia
The approach to therapy largely depends on kidney function. If kidney function is normal, stopping the source of magnesium will promptly restore normal plasma magnesium concentrations. If necessary, loop or thiazide diuretics can be used to increase renal excretion of magnesium. In patients with mild-to-moderate degrees of either chronic or acute kidney impairment (eGFR between 15 and 45 mL/min/1.73 m 2 ), urinary elimination of magnesium may be limited. Again, the initial treatment begins with removing magnesium-containing medications followed by administering intravenous isotonic fluids such as saline plus a loop diuretic. In patients with end-stage kidney failure (eGFR less than 15 mL/min/1.73 m 2 , dialysis may be the only option ( ) .
Proper collection and handling of samples
See Chapter 10 .
Diagnosis of magnesium abnormalities
While measurement of total magnesium concentrations in serum remains the usual diagnostic test for the detection of magnesium abnormalities, total magnesium concentrations have two limitations. First, about 30% of magnesium is protein bound. Therefore, as with total versus ionized calcium, total magnesium may not reflect the physiologically active ionized magnesium. Second, and probably of greater significance, because magnesium is primarily an intracellular ion, concentrations of either total or ionized magnesium in blood will not necessarily reflect the intracellular and overall body stores of magnesium. Symptomatic hypomagnesemia may not appear until serum total magnesium levels fall below 0.5 mmol/L (well below the typical reference limit), which make associations between serum magnesium concentrations and the benefit of treatment difficult ( ) .
The magnesium retention test assesses overall magnesium status, but its use may be restricted to special circumstances when there is a strong suspicion of magnesium deficiency despite a normal serum magnesium. A magnesium retention test requires over 48 h to complete, collection of two 24-h urines, and giving 30 mmol of MgSO 4 intravenously over 12 h. If more than 60%–70% of Mg is excreted in the urine following an intravenous load, Mg deficiency is unlikely, while magnesium-deficient patients excrete less than 50% of the administered magnesium ( , ) . Normal renal handling of Mg is necessary for this test to be useful because increased magnesium losses due to diabetes, medications or alcohol ingestion may result in a false-negative test. If kidney function is compromised, one may see false-positive results, so the Mg retention test should not be used in patients with renal impairment or in transplant patients receiving cyclosporine or tacrolimus, both of which cause urinary Mg wasting ( ) .
Ionized Mg versus total Mg
The routine measurement of ionized magnesium has been available for many years since sensors for magnesium ion electrodes were developed from lengthy studies on over 200 ionophores produced by the late Dr. Wilhelm Simon and his colleagues ( ) . However, ionized magnesium measurements have more analytical variability than ionized calcium measurements because all Mg ion-sensitive electrodes are also sensitive to Ca ions. While this interference by Ca ion is corrected by simultaneous measurement of the Ca ion concentration, this additional test adds variability to the ionized magnesium result ( ) .
There are also a few studies relating intracellular levels of magnesium to clinical information. For example, one study concluded that red blood cell magnesium was the best parameter to measure because it gave a higher incidence of hypomagnesemia (37%) than did either ionized magnesium (22%) or total magnesium (16%) ( ) . However, regardless of its possible clinical value, at least for the foreseeable future, these measurements are not practical for clinical use.
While some studies conclude that measurements of ionized magnesium improve clinical accuracy, others find that either total or ionized magnesium provides about the same clinical value in detecting hypomagnesemia. Conclusions are often based on whether the total serum magnesium and ionized Mg do or do not correlate well in various disease states.
While it is logical to equate the physiologic activity of ionized Mg with better clinical reliability, there are other issues to consider. Total magnesium measurements have better analytical reliability and have far greater familiarity in clinical practice. However, because ionized magnesium measurements are on “blood gas” analyzers, they have the advantage of faster turnaround times and can be used in point-of-care settings. Hopefully, ionized magnesium will become more widely used and gain clinical familiarity.
Here are representative reports that conclude the total and ionized Mg results give equivalent clinical information.
Saha et al. reported strong correlations between total and ionized Mg concentrations in serum from hemodialysis patients, and in patients with intestinal disease, alcoholic liver disease, and chronic renal disease ( , ) .
Lanzinger et al. often found that, in patients during abdominal surgery who did not have massive transfusions, the changes in ionized and total serum Mg concentrations correlated closely ( ) . Even though total serum Mg slightly overestimated the prevalence of hypomagnesemia, it adequately screened for hypomagnesemia.
Koch et al. found a strong correlation between ionized and total Mg ( r = 0.90), with hypomagnesemia in 21% of ICU patients measured by ionized Mg and 18% of ICU patients measured by total Mg. They concluded that ionized Mg could be inferred from total Mg ( ) .
In a study on 286 patients of indigenous and nonindigenous Australian descent, with or without diabetes, Longstreet and Vick noted significant correlations between total and ionized magnesium measurements in all groups ( r = 0.75; P < .001). Among people with diabetes, the correlation coefficient ( r ) was 0.81 whereas in nondiabetics, the r was 0.66. Based on the strong correlation between total and ionized Mg concentrations in serum, they concluded that either gives an accurate assessment of magnesium status in health and chronic diabetes, irrespective of ethnicity ( ) .
Here are representative reports that conclude that ionized Mg measurements provide better clinical information.
A poor correlation between ionized and total Mg was noted in critically ill patients in studies by both Barrera et al. ( r = 0.57) ( ) , and by Johansson and Whiss (r = 0.59) ( ) . However, after magnesium supplementation, both ionized and total Mg increased: ionized by 0.05 mmol/L and total by 0.11 mmol/L ( ) .
Fiser et al. observed that ionized magnesium was decreased in many critically ill pediatric patients who had normal total magnesium concentrations ( ) . In this study, the use of albumin-corrected total magnesium was not reliable in estimating the ionized magnesium.
In patients undergoing cardiopulmonary bypass surgery, plasma ionized (but not total) magnesium was decreased significantly by 24 h after bypass ( ) .
In the report by Yeh et al. ( ) the ionized magnesium result was assumed to always be “correct.” For example, if any magnesium supplementation was given based on a low total magnesium (34 patients), it was called “inappropriate administration” in 28 of the patients who had normal ionized Mg results. The 28 with low total Mg but normal ionized Mg were considered erroneously low, and resulted in 27 additional and presumably unnecessary repeat Mg measurements and administration of 60 g of MgSO 4 .
Variable correlations were reported by Hutton et al. ( ) . In the general ICU population, they reported a strong correlation (r = 0.95) between ionized and total Mg, and 144 of the 185 samples fell in the same category (hypo-, normo-, or hypermagnesemia) for both ionized and total Mg based on their corresponding reference intervals of 0.49–0.71 mmol/L and 0.70–1.0 mmol/L, respectively. Also, the regression slope of 0.71 corresponded with 70% of magnesium being in the ionized form. However, in citrate-anticoagulated patients treated with continuous venovenous hemofiltration (CVVH), half of these patients showed normal total Mg and decreased ionized Mg. Based on this, they concluded that ionized Mg was better than total Mg as a marker for hypomagnesaemia in these CVVH patients.
The results from the study by Wilkes et al. could be interpreted to favor either ionized or total Mg measurements. They emphasized that correcting the ionized Mg by magnesium supplementation during cardiopulmonary bypass (CPB) reduced postoperative risks. However, their figures show that both total and ionized Mg declined almost identically during CPB and increased almost identically when magnesium supplementation was given to correct the hypomagnesemia ( ) .
To conclude the discussion of whether total or ionized magnesium measurement is more clinically appropriate, Longstreet and Vink provided an interesting theory ( ) . The chronic or acute nature of the patients’ condition may be relevant. In most chronic conditions, a generalized magnesium deficiency would maintain a consistent decline in both the total and ionized magnesium pools. However, in acute conditions that often require critical care, the rapid onset could rapidly alter the binding of Mg ions in serum and possibly cause greater relative differences between total and ionized magnesium concentrations. Thus, the chronic or acute nature of the disease process may be most relevant in deciding to measure total or ionized Mg to assess magnesium status.
Reference intervals for magnesium
Reference intervals (ranges) for total and ionized magnesium are shown in Table 6.4 , and are based on literature sources. For ionized Mg, the lower limit of the reference intervals varied from 0.43 to 0.49 mmol/L and the upper limit varied from 0.59 to 0.71 mmol/L ( , , ). For total Mg, the lower limits varied from 0.64 to 0.76 mmol/L and the upper limits varied from 0.90 to 1.10 mmol/L ( , , , , , , ) . Concentrations of magnesium in serum are slightly higher in older children and adults.
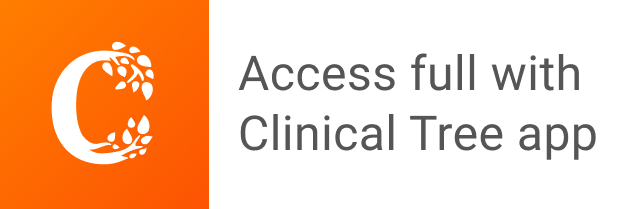