Local anesthesia can be defined as loss of sensation in a discrete region of the body caused by disruption of impulse generation or propagation. Local anesthesia can be produced by various chemical and physical means. However, in routine clinical practice, local anesthesia is produced by several compounds whose mechanism of action is similar, although they have different durations of action, and from which recovery is normally spontaneous, predictable, and complete.
History
Clinical use of local anesthetics began with cocaine in the 1880s. The topically applied local anesthetic benzocaine and the injectable drugs procaine, tetracaine, and chloroprocaine were subsequently developed as adaptations of cocaine’s structure as an amino ester ( Figs. 10.1 and 10.2 ).


In 1948, lidocaine was introduced as the first member of a new class of local anesthetics, the amino amides. Advantages of the amino amides over the earlier amino esters included more stability and a reduced frequency of allergic reactions. Because of these favorable properties, lidocaine became the template for the development of a series of amino-amide anesthetics (see Fig. 10.2 ).
Along with lidocaine, most amino-amide local anesthetics are derived from the aromatic amine xylidine, including mepivacaine, bupivacaine, ropivacaine, and levobupivacaine. Ropivacaine and levobupivacaine share an additional distinctive characteristic: they are single enantiomers rather than racemic mixtures. They are products of a developmental strategy that takes advantage of the differential stereoselectivity of neuronal and cardiac sodium ion channels in an effort to reduce the potential for cardiac toxicity (see “ Adverse Effects ”). Almost all of the amides undergo biotransformation in the liver, whereas the esters undergo hydrolysis in plasma.
Nerve Conduction
Under normal or resting circumstances, the neural membrane is characterized by a negative potential of roughly –90 mV (the potential inside the nerve fiber is negative relative to the extracellular fluid). This negative potential is created by energy-dependent outward transport of sodium and inward transport of potassium ions, combined with greater membrane permeability to potassium ions relative to sodium ions. With excitation of the nerve, there is an increase in the membrane permeability to sodium ions, causing a decrease in the transmembrane potential. If a critical potential is reached (i.e., threshold potential), there is a rapid and self-sustaining influx of sodium ions resulting in a propagating wave of depolarization, the action potential, after which the resting membrane potential is reestablished.
Nerve fibers can be classified according to fiber diameter, presence (type A and B) or absence (type C) of myelin, and function ( Table 10.1 ). The nerve fiber diameter influences conduction velocity; a larger diameter correlates with more rapid nerve conduction. The presence of myelin also increases conduction velocity. This effect results from insulation of the axolemma from the surrounding media, forcing current to flow through periodic interruptions in the myelin sheath (i.e., nodes of Ranvier) ( Fig. 10.3 ).
Fiber | Diameter (μm) | Conduction Velocity (m/sec) | Function | |
---|---|---|---|---|
Type | Subtype | |||
A (myelinated) | Alpha | 12-20 | 80-120 | Proprioception, large motor |
Beta | 5-15 | 35-80 | Small motor, touch, pressure | |
Gamma | 3-8 | 10-35 | Muscle tone | |
Delta | 2-5 | 5-25 | Pain, temperature, touch | |
B (myelinated) | 3 | 5-15 | Preganglionic autonomic | |
C (unmyelinated) | 0.3-1.5 | 0.5-2.5 | Dull pain, temperature, touch |

Local Anesthetic Actions On Sodium Channels
Local anesthetics act on a wide range of molecular targets, but they exert their predominant desired clinical effects by blocking sodium ion flux through voltage-gated sodium channels. Voltage-gated sodium channels are complex transmembrane proteins comprising large alpha subunits and much smaller beta subunits ( Fig. 10.4 ).

The alpha subunits have four homologous domains arranged in a square, each composed of six transmembrane helices, and the pore lies in the center of these four domains. Beta subunits modulate electrophysiologic properties of the channel and they also have prominent roles in channel localization, binding to adhesion molecules, and connection to intracellular cytoskeletons. There are nine major subtypes of sodium channel alpha subunits in mammalian tissues and four major subtypes of beta subunits.
Different sodium channel subtypes are expressed in different tissues, at diverse developmental stages, and in a range of disease states. Sodium channel subtypes are an active area of investigation around human diseases with spontaneous pain and pain insensitivity, as targets of new analgesics, and in other areas of medicine, including cardiology and neurology. Sodium channel subtypes will be discussed briefly again later in this chapter (see “ When Local Anesthesia Fails ” and “ Future Local Anesthetics ”).
From an electrophysiologic standpoint, local anesthetics block conduction of impulses by decreasing the rate of depolarization in response to excitation, preventing achievement of the threshold potential. They do not alter the resting transmembrane potential, and they have little effect on the threshold potential.
Sodium channels cycle between resting, open, and inactive conformations. During excitation, the sodium channel moves from a resting closed state to an open activated state, with an increase in the inward flux of sodium ions and consequent depolarization. The channel transitions to an inactive state and must undergo further conformational change back to a resting state before it can again open in response to a wave of depolarization.
According to the modulated receptor model, local anesthetics act not by physically “plugging the pore” of the channel but rather by an allosteric mechanism; that is, by changing the relative stability and kinetics of cycling of channels through resting, open, and inactive conformations. In so doing, the fraction of channels accessible to opening and conducting inward sodium currents in response to a wave of depolarization is reduced. This mechanism provides nerve blocks that are either a “use-dependent” or “frequency-dependent” type of block; that is, the block intensifies with more frequent rates of nerve firing.
pH, Net Charge, and Lipid Solubility
The predominant binding site for local anesthetics on sodium channels is near the cytoplasmic side of the plasma membrane. A major structural requirement for a molecule to be an effective local anesthetic is sufficient solubility and rapid diffusion in both hydrophilic environments (extracellular fluid, cytosol, and the headgroup region of membrane phospholipids) and in the hydrophobic environment of the lipid bilayers in plasma membranes.
The amino-amide and amino-ester local anesthetics in common clinical use achieve this aim of good solubility in both water and fat because they each contain a tertiary amine group that can rapidly convert between a protonated hydrochloride form (charged, hydrophilic) and an unprotonated base form (uncharged, hydrophobic). The charged, protonated form is the predominant active species at binding sites on sodium channels ( Fig. 10.5 ).

The relative proportion of charged and uncharged local anesthetic molecules is a function of the dissociation constant of the drug and the environmental pH. Recalling the Henderson-Hasselbalch equation, the dissociation constant (Ka) can be expressed as follows:
pKa=pH−log([base]/[conjugate acid])
If the concentrations of the base and conjugate acid are equal, the latter component of the equation cancels (because log 1 = 0). Thus, the pKa provides a useful way to describe the propensity of a local anesthetic to exist in a charged or an uncharged state. The lower the pKa, the greater is the percent of un-ionized fraction at a given pH. In contrast, because the pKa values of the commonly used injectable anesthetics are between 7.6 and 8.9, less than one half of the molecules are un-ionized at physiologic pH ( Table 10.2 ). The base forms of local anesthetics are poorly soluble in water and less stable, so they are generally marketed as water-soluble hydrochloride salts at slightly acidic pH. Bicarbonate is sometimes added to local anesthetic solutions immediately before injection to increase the un-ionized fraction in an effort to hasten the onset of anesthesia. Other conditions that lower pH, such as tissue acidosis produced by infection, inflammation, or ischemia, may likewise have a negative impact on the onset and quality of local anesthesia.
Classification and Compounds | pK a | % Nonionized at pH 7.4 | Potency a | Max. Dose (mg) for Infiltration b | Duration After Infiltration (min) | Topical | Local | IV | Periph | Epi | Spinal |
---|---|---|---|---|---|---|---|---|---|---|---|
Esters | |||||||||||
Procaine | 8.9 | 3 | 1 | 500 | 45-60 | No | Yes | No | Yes | No | Yes |
Chloroprocaine | 8.7 | 5 | 2 | 600 | 30-60 | No | Yes | Yes | Yes | Yes | Yes c |
Tetracaine | 8.5 | 7 | 8 | Yes | Yes d | No | No | No | Yes | ||
Amides | |||||||||||
Lidocaine | 7.9 | 24 | 2 | 300 | 60-120 | Yes | Yes | Yes | Yes | Yes | Yes c |
Mepivacaine | 7.6 | 39 | 2 | 300 | 90-180 | No | Yes | No | Yes | Yes | Yes c |
Prilocaine | 7.9 | 24 | 2 | 400 | 60-120 | Yes e | Yes | Yes | Yes | Yes | Yes c |
Bupivacaine, levobupivacaine | 8.1 | 17 | 8 | 150 | 240-480 | No | Yes | No | Yes | Yes | Yes |
Ropivacaine | 8.1 | 17 | 6 | 200 | 240-480 | No | Yes | No | Yes | Yes | Yes |
a Relative potencies vary based on experimental model or route of administration.
b Dosage should take into account the site of injection, use of a vasoconstrictor, and patient-related factors.
c Use of procaine, lidocaine, mepivacaine, prilocaine, and chloroprocaine for spinal anesthesia is somewhat controversial; indications are evolving (see text).
d Used in combination with another local anesthetic to increase duration.
Lipid solubility of a local anesthetic affects tissue penetration, time course of uptake, potency, and duration of action. Duration of the local anesthetic action also correlates with protein binding, which likely serves to retain anesthetic within the nerve.
Degrees of anesthetic potency may be altered by the in vitro or in vivo system in which these effects are determined. For example, tetracaine is approximately 20 times more potent than bupivacaine when assessed in isolated nerve, but these drugs are nearly equipotent when assessed in vivo. Even when assessed in vivo, comparisons among local anesthetics may vary based on the specific site of application (spinal versus peripheral block) because of secondary effects such as the inherent vasoactive properties of the anesthetic.
Differential Local Anesthetic Blockade
From a clinical viewpoint and from electrophysiologic measurements, local anesthesia is not an all-or-none phenomenon: patients experience gradations in the intensity of sensory and motor blockade that vary over time following local anesthetic injections. Clinically apparent “numbness” generally correlates with intraneural concentrations of local anesthetics but also reflects complex integration and processing of inputs in the spinal dorsal horn and at supraspinal sites in the somatosensory pathway. When compound action potentials are recorded in peripheral nerves exposed to local anesthetics in varying concentrations and lengths of nerve exposed, conduction blockade is facilitated either by increasing the concentration of local anesthetic or by increasing the length of nerve exposed to more dilute concentrations. At the limit of short lengths of nerve exposed to local anesthetic, conduction blockade requires exposure of at least three successive nodes of Ranvier to prevent the action potential from “skipping over” the region of local anesthetic exposure.
Historically, the term differential blockade in clinical textbooks referred to the observation that infusions of dilute concentrations of local anesthetic could produce analgesia and signs of autonomic blockade with relative sparing of motor strength. This clinical trend is not readily explained by the electrophysiologic observations of action potential blockade in large and small fibers perfused to steady state. The mechanisms underlying this divergence between clinical experience and experimental data are poorly understood, but they may be related to the anatomic and geographic arrangement of nerve fibers, variability in the longitudinal spread required for neural blockade, effects on other ion channels, and inherent impulse activity.
Spread of Local Anesthesia After Injection
When local anesthetics are deposited around a peripheral nerve, they must cross a series of diffusion barriers to access sodium channels in nerve axons ( Fig. 10.6 ). With large nerve trunks, they diffuse from the outer surface (mantle) toward the center (core) of the nerve along a concentration gradient ( Fig. 10.7 ). As a result, nerve fibers located in the mantle of the mixed nerve are blocked first. These mantle fibers are generally distributed to more proximal anatomic structures, whereas distal structures are innervated by fibers near the core. This anatomic arrangement accounts for the initial development of proximal anesthesia with subsequent distal involvement as local anesthetic diffuses to reach more central core nerve fibers. Skeletal muscle weakness may precede sensory blockade if the motor nerve fibers are more superficial. The sequence of onset and recovery from conduction blockade of sympathetic, sensory, and motor nerve fibers in a mixed peripheral nerve depends as much or more on the anatomic location of the nerve fibers within the mixed nerve as on their intrinsic sensitivity to local anesthetics.
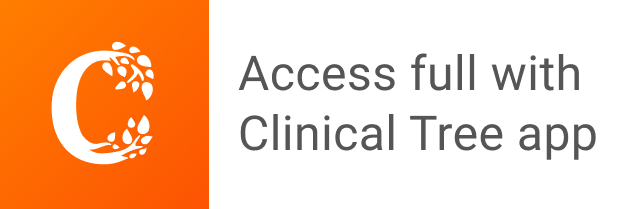