Local anesthetics have been used for centuries and represent some of the oldest drugs in the armamentarium of anesthesiologists. The story of the coca leaf and its alkaloid derivative, cocaine, is the best-known example of this. Members of the pre-Columbian Inca civilization would chew coca leaves to reduce pain and for its stimulant effects. Coca leaves are still chewed and brewed in teas in present day South American cultures. Research has found traces of coca in mummies from over 3000 years ago.1 It is believed that shamans in the Incan culture would chew coca leaves and that the mixture of juice and saliva would be spit into the surgical site during trepanning operations. Eventually, coca was farmed for exportation to Europe starting in the 16th century. Coca wines, a blend of wine and cocaine, were popularized in the mid-1800s in Europe and eventually made an appearance in America. However, with the onset of prohibition in America, the wine in coca wine was replaced with a syrup, which became the basis for the first recipe of Coca-Cola. During the period of coca wine production in Europe, a German chemist named Albert Niemann in 1859 became the first person to isolate the primary alkaloid of coca, which he termed cocaine.2 The first clinical use of cocaine was as an ophthalmic anesthetic by Karl Koller in 1884. Sigmund Freud, Koller’s colleague, had done extensive testing of cocaine and had noted its anesthetic qualities prior to Koller’s clinical use. Ultimately, the addictive properties of cocaine were recognized, and an effort was made to synthesize other local anesthetics. Einhorn developed procaine for clinical use in 1905, which became the local anesthetic of choice. Cocaine is still used even today (mostly for head, neck, and ophthalmic procedures), but its popularity is waning in the medical field. Unfortunately, most cocaine use today is as an illegal drug via snorting, smoking, or injection.
Numerous ester and amide formulations have been produced, starting in the 1890s. There was a push in the early to mid-1900s to improve the pharmacokinetics and toxicity profile of local anesthetics. Lidocaine is often considered to be the prototypical local anesthetic, and it was first used clinically in 1944 by Swedish chemist Nils Lofgren and patented in 1948.3 Bupivacaine, another popular local anesthetic in common use today, was first synthesized in 1957 and introduced clinically in 1965. Clinicians have appreciated bupivacaine for its long duration of action but also recognized its troublesome cardiovascular toxicity profile and difficult resuscitation following inadvertent intravenous injection. In response to this, ropivacaine was developed and introduced clinically in 1996.4 Bupivacaine consists of a racemic mixture of both S and R stereoisomers. However, ropivacaine consists of a single S stereoisomer, which shows lower potency at cardiac sodium channels and only slightly decreased anesthetic potency.
There will continue to be further research and development into the production of an ideal local anesthetic. However, one should consider that the central nervous system (CNS) and cardiovascular toxicities seen with use of a local anesthetic are in fact an extension of the drug’s normal mechanism of action to undesired sites. For this reason, a perfect local anesthetic without toxicity might not be possible. Also, as local anesthetics are now being used more often via continuous infusions, and for chronic pain and cancer, development is underway to find ways to increase the duration of action via liposomal encapsulation and microspheres for sustained release.5,6,7, and 8 Transcutaneous lidocaine delivery systems are already in routine use in many chronic pain clinics.
Normal resting nerve membrane potential is –70 to –60 mV. This negative potential of the nerve interior is maintained by an energy-dependent Na+/K+ pump, primarily via potassium disequilibria (Figure 12–1). With the arrival of an appropriate nerve stimulus, the membrane becomes less negative, ultimately reaching an activation threshold of approximately –55 mV. At this point, sodium channels are converted from their resting to an opened configuration, followed by a fast inward sodium flow down its concentration gradient to depolarize the membrane to approximately +40 mV (Figure 12–2). This depolarization creates a positive feedback loop of increased sodium permeability and inward sodium current that encourages further depolarization and downstream propagation of the stimulus. The sodium channels will eventually close spontaneously to an inactivated state and potassium channels will open with an outward flow of potassium down its concentration gradient. These 2 events will shift the process toward local repolarization of the membrane. To accomplish repolarization, there is first a hyperpolarization of the membrane immediately following an impulse that causes a refractory period during which sodium channels remain inactivated, potassium channels are still activated, and a nerve impulse cannot be conducted (see Figure 12–1). This concept explains the unidirectional aspect of nerve impulse propagation along the axon; an impulse cannot reverse direction and spread retrograde because the region behind the impulse is refractory to further stimulation. Eventually, sodium channels switch from inactivated to their resting configuration and potassium channels close, repolarizing the cell to its resting membrane potential. The Na+/K+ ATP-ase maintains this repolarized, resting state.
Figure 12–1
Schematic drawing of sodium (Na+)/potassium (K+) pump. The energy-dependent process transports sodium ion from intracellular cytoplasm to extracellular fluid, while at the same time transporting potassium ion from the extracellular fluid to the intracellular cytoplasm. ADP, adenosine diphosphate; ATP, adenosine triphosphate.

Figure 12–2
Schematic idealized plot of membrane voltage change during an action potential in response to a stimulus. Text labels represent the 4 phases (rising, peak, falling, and undershoot) and refractory period of the action potential. The refractory period may cross over more than one of the phases. ms, milliseconds; mV, millivolts.

The goal of local anesthesia is to induce a reversible loss of nociception within a specific region of the body. Local anesthetics accomplish this by reversibly blocking nerve impulse conduction by binding to sodium channels and inhibiting sodium influx. Local anesthetics do not affect the resting membrane potential. Instead, the local anesthetic molecule binds various receptor sites on the sodium channel, preventing sodium influx. As discussed above, there are 3 distinct channel configurations during the cycle of depolarization and repolarization: resting, open, and inactivated. The open and inactivated channel conformations bind local anesthetics better than the resting state. A weak interaction can occur between a local anesthetic molecule and the resting channel that will prevent a change to the open state—this is referred to as a tonic or resting inhibition. Nerves that are actively and repeatedly being depolarized have more of their channels in an open or inactivated state, and these nerves are therefore more susceptible to blockade by local anesthetics—this is referred to as frequency-dependent (phasic) inhibition.
Several mechanisms of local anesthetic mechanism have been proposed. It is thought that the local anesthetic molecule interacts with a binding site on the intracellular side of the sodium channel. This means that the drug must traverse the bilipid membrane to gain entry to the cell interior, and a neutral form of the drug is required to easily cross the membrane. It is also thought that local anesthetic molecules might interfere with neuronal membrane fluidity. The drug is able to expand the membrane surface and effectively close the sodium channel, thereby preventing ion passage.
The 2 available structural classes of local anesthetics include aminoesters and aminoamides (Figure 12–3). A lipophilic aromatic ring is connected to an ionizable, hydrophilic tertiary amine via either the ester or amide link. Various substitutions on these 3 portions create the wide variety of local anesthetics available today.
All local anesthetics currently used in clinical practice are weak bases, and the tertiary amine portion of the molecule can be in varying states of protonation depending on the surrounding environment’s pH. Understanding the relationship between the charged and uncharged form is key to understanding the clinical effect of local anesthetics.
Looking at Table 12–1, the pKa for most clinically available local anesthetics is in the range of 7.7 to 8.9, meaning that when exposed to normal body pH of 7.4, more local anesthetic molecules will exist in the charged, ionized form. The degree of local anesthetic ionization is important, as the ionized form is active at the sodium channel receptor site and does not readily dissociate from the channel, but is also poorly lipid soluble. In contrast, the neutral form is more lipid soluble and can easily traverse nerve membranes to the site of action. Early studies of local anesthetics proved that a local anesthetic could more effectively block nerve transmission if the surrounding pH was more alkaline, as more molecules would be in a neutral state and able to penetrate the membrane to successfully bind the sodium channel.9 This concept is also seen clinically as local anesthetics injected into an area of inflamed or infected tissue (with a lower pH) will not be as effective. This also explains why clinicians may choose to add bicarbonate to their local anesthetic solutions prior to injection, in an attempt to increase the pH of the local anesthetic solution, thereby increasing the proportion of uncharged molecules available. There are a lack of good data to support alkalinization of local anesthetic solutions, although it is commonly done. Some believe that along with speeding the onset time, this practice also decreases the pain patients may experience upon injection of the local anesthetic.
pKa | % Protein Binding | Speed of Onset | Duration of Action (hours) | |
---|---|---|---|---|
Amides | ||||
Lidocaine (Xylocaine) | 7.8 | 70 | Rapid | 1–2 |
Bupivacaine (Marcaine, Sensorcaine) | 8.1 | 96 | Slow | 4–8 |
Levobupivacaine | 8.1 | 96 | Sow | 4–8 |
Ropivacaine (Naropin) | 8.1 | 94 | Slow | 4–8 |
Mepivacaine (Carbocaine) | 7.6 | 78 | Slow | 1.5–3 |
Prilocaine (Novocaine) | 7.8 | 55 | Slow | 1–2 |
Esters | ||||
Procaine (Citanest) | 8.9 | 6 | Slow | 1 |
Chloroprocaine (Nesacaine) | 9.0 | 7 | Rapid | 0.5 |
Tetracaine, Amethocaine (Pontocaine) | 8.2 | 75 | Slow | 3 |
Benzocaine (Cetacaine) | 2.5 | |||
Cocaine | 8.7 |
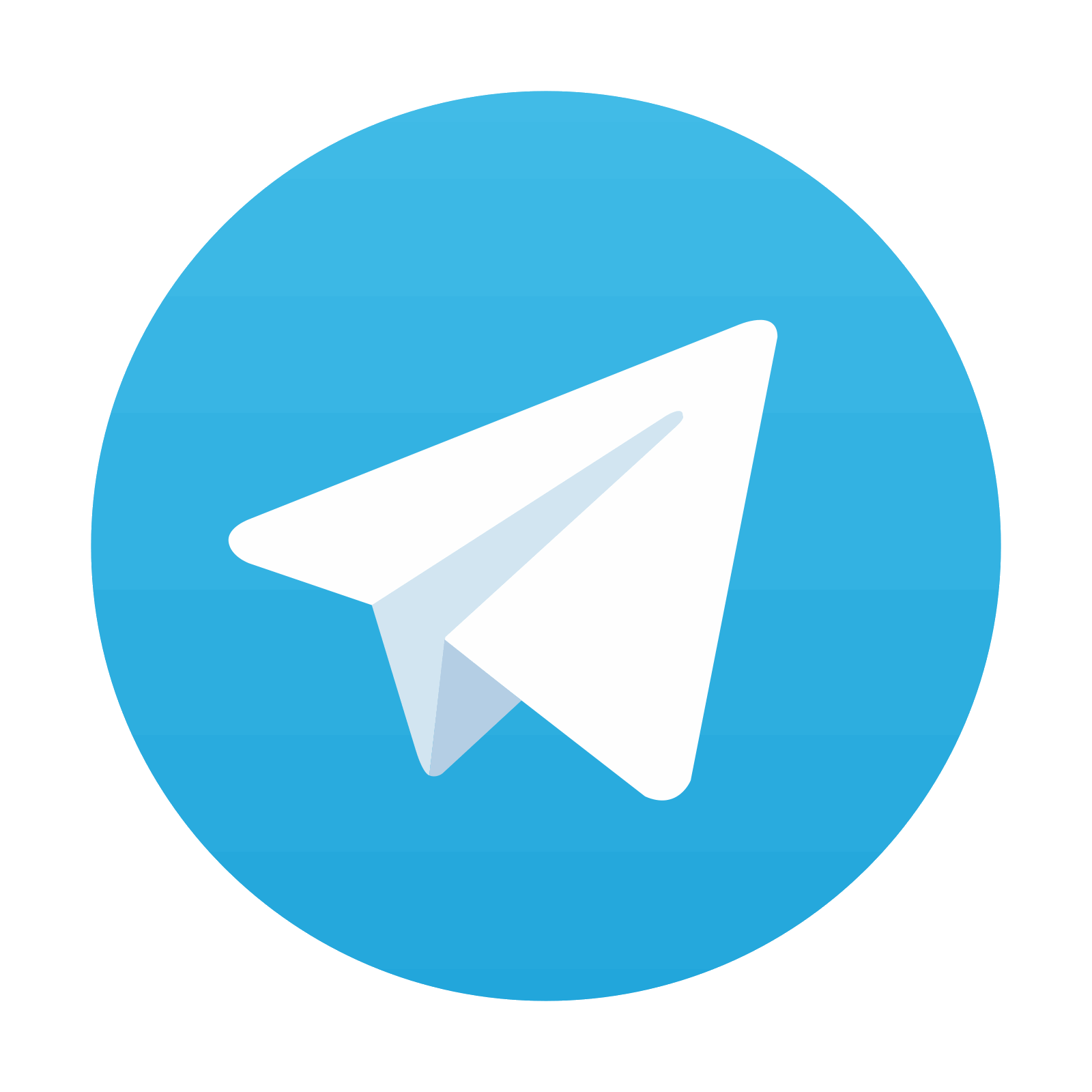
Stay updated, free articles. Join our Telegram channel
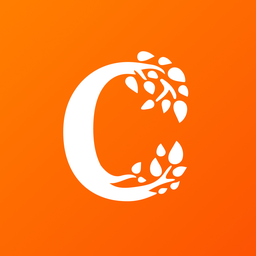
Full access? Get Clinical Tree
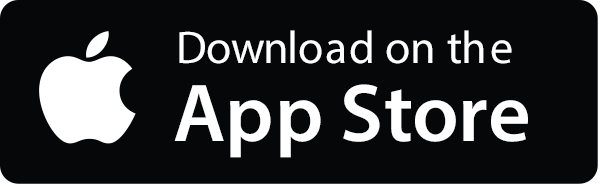
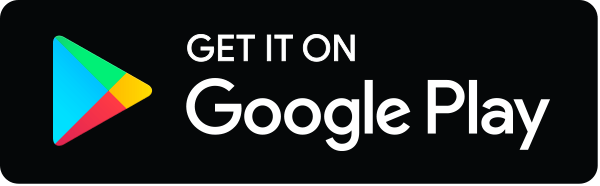
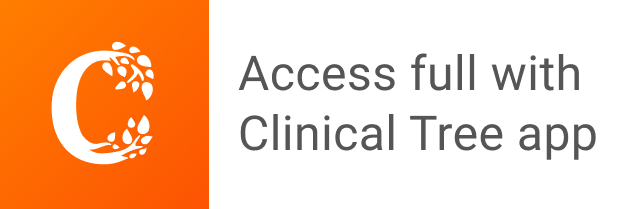