Local Anesthetic Systemic Toxicity
Graeme A. McLeod
John F. Butterworth IV
J. A. W. Wildsmith
Systemic toxicity is the term used to describe the clinical symptoms associated with extreme plasma concentrations of local anesthetics. Most reactions involve the central nervous system (CNS) first, with cardiovascular collapse being secondary to hypoxia consequent upon central respiratory depression. However, primary cardiovascular complications can occur under certain circumstances, most commonly the rapid, but accidental, intravascular injection of a large dose of drug, particularly one of the longer-acting agents. The drugs vary in their potential for causing systemic toxicity; the general view of the relative risk of this complication is shown in Figure 4-8 and clearly relates to local anesthetic potency. Although some variation in early symptoms exists between individuals, the actual features of a toxic reaction are drug nonspecific and generally demonstrate the consequences of progressive CNS depression, as noted in Figure 5-1. Classically, the initial features include perioral numbness or paresthesia, light-headedness, tinnitus, dysarthria, visual disturbances, and muscular twitching. This may be followed by convulsions, coma, respiratory arrest and, finally, cardiovascular depression. Cardiovascular features may include heart block, hypotension, bradycardia, ventricular arrhythmia (such as torsade de pointes), and cardiac arrest. Resuscitation from local anesthetic–induced cardiac arrest is extremely difficult, and has often been unsuccessful.
Such a major, life-threatening reaction can occur as the result of a simple, but very significant, overdose with a correctly placed injection. Excessive dosing during a continuous block is a more likely cause, although the many factors that can affect systemic absorption (Chapter 3) may interact to cause occasional problems with otherwise appropriate doses. However, most severe reactions are the result of unusually rapid increases in plasma concentration. Inflammation at the site of injection may contribute to this by speeding absorption, but the accidental intravascular injection of significant amounts of drug is the more likely cause, with sudden cuff deflation during intravenous (IV) regional anesthesia being a block-specific variation. It will not escape notice that all of these factors are subject to some degree of operator control and, given that the reactions are extremely difficult to treat, the overall message must be that prevention is better than cure (see Fig. 4-8).
Historical Background
Systemic toxicity secondary to local anesthetics was recognized historically, but only as the stereotypical progression from prodromal symptoms to convulsions to circulatory collapse illustrated in Figure 4-8. However, in 1979, an editorial by Albright (1) described six cases of cardiac arrest without preceding CNS symptoms and made the claim that these were due to inadvertent intravascular injection of bupivacaine or etidocaine. Four years later, at a meeting of the U.S. Food and Drug Administration (FDA), Albright presented a total of 53 cases of prolonged and difficult resuscitation after inadvertent intravascular injection of bupivacaine or etidocaine, with death being the outcome in 31. An important feature was that 24 out of 35 pregnant women died (69%) of systemic reactions following an epidural block, whereas only seven out of 18 nonpregnant patients (39%) died (the latter group had received a variety of regional techniques). The outcome from the FDA meeting was that 0.75% bupivacaine was no longer recommended for obstetric anesthesia, despite having being used in only 27 (51%) of the reported patients, another eight (15%) having received the 0.5% preparation.
Ironically, the introduction of bupivacaine into anesthetic practice in the 1960s had done much to develop the subspecialty of obstetric anesthesia. Compared to lidocaine, bupivacaine caused less cumulative toxicity because of a more convenient duration of action and did not require epinephrine to limit systemic absorption or prolong its effect. Further clinical benefits of epidural bupivacaine include a greater degree of differential sensory block (that is, analgesia with relative sparing of motor function) and limited transfer of local anesthetic to the fetus. In these circumstances, it was not surprising that anesthesiologists did not wish to dispense with bupivacaine, but sought to alter anesthetic practice. Greater attention to the use of test doses (both of local anesthetics and other markers of intravascular injection), careful aspiration (especially of the epidural catheter) before injection, and incremental injection (dividing the total dose into 4- to 5-mL aliquots and injecting these at 45- to 60-second intervals) were partially successful in reducing the incidence of systemic toxicity. However, not even the use of all these techniques seems to be able to prevent the occasional accidental intravascular injection, and cases of systemic toxicity have continued to occur in different clinical settings.
The incidence of systemic toxicity reported in several observational studies performed during the last quarter of a century or so is summarized in Table 5-1. The most obvious feature is that the overall incidence of convulsions from all types of regional block has reduced dramatically—from 1 in 518 in 1978 to 1 in 4,510 in 1997, and to 1 in 17,564 in 2002. However, analysis of these data according to the site of injection (either neuraxial or major limb block) reveals a rather more disturbing picture. The incidence of convulsions secondary to limb block varied from 1 in 502 to 1 in 8,371, between four and sixteen times greater than the incidence of convulsions associated with epidural block. In one series, for example, Brown
et al. (2) recorded eight convulsions in 6,620 axillary blocks (1 in 827) and seven convulsions in 912 interscalene/supraclavicular blocks (1 in 130), suggesting that nerve block above the clavicle is particularly hazardous, even when compared with other types of brachial plexus block, let alone epidural anesthesia (1 in 8,435). Fortunately, none of the 58 patients suffering a convulsion in the combined series of Brown (2) and Auroy (3,4) progressed to cardiac collapse.
et al. (2) recorded eight convulsions in 6,620 axillary blocks (1 in 827) and seven convulsions in 912 interscalene/supraclavicular blocks (1 in 130), suggesting that nerve block above the clavicle is particularly hazardous, even when compared with other types of brachial plexus block, let alone epidural anesthesia (1 in 8,435). Fortunately, none of the 58 patients suffering a convulsion in the combined series of Brown (2) and Auroy (3,4) progressed to cardiac collapse.
![]() Figure 5-1. Relationship of signs and symptoms of local anesthetic toxicity to plasma concentrations of lidocaine. |
It might be argued that such reactions are the consequence of poor clinical practice, but this position is confounded by considering the results from controlled clinical trials conducted for approval of newer, safer local anesthetics. One of the consequences of cardiac toxicity following bupivacaine was the search for safer long-acting agents. Two such agents, ropivacaine (Astra-Zeneca, Sweden) and levobupivacaine (Abbott, Chicago), underwent a rigorous series of phase III studies for the FDA and the European regulatory authorities before being licensed for clinical practice. Data gathered in regulatory studies such as these provide detailed descriptions of the clinical management of the patients and any adverse events, particularly their relationship to systemic toxicity (5,6). All of the prospective, randomized, ethically approved studies were undertaken in hospitals noted for their expertise in regional anesthesia, and the blocks were performed by experienced staff, such as consultants or dedicated research fellows.
Table 5-1 Incidence of convulsions in large-scale studies of regional anesthesia: epidural and peripheral block | |||||||||||||||||||||||||||||||||||||||||||||||||||||||||||||||||||||||||||||||||||||||||
---|---|---|---|---|---|---|---|---|---|---|---|---|---|---|---|---|---|---|---|---|---|---|---|---|---|---|---|---|---|---|---|---|---|---|---|---|---|---|---|---|---|---|---|---|---|---|---|---|---|---|---|---|---|---|---|---|---|---|---|---|---|---|---|---|---|---|---|---|---|---|---|---|---|---|---|---|---|---|---|---|---|---|---|---|---|---|---|---|---|
|
In spite of that high level of care, nine adults out of approximately 4,500 patients were identified as experiencing systemic toxicity secondary to accidental IV administration of local anesthetic (seven women, two men). Three patients were pregnant and scheduled for Cesarean section. Six patients received ropivacaine, two bupivacaine, and one patient levobupivacaine. Seizures occurred in the two patients undergoing upper limb block (one ropivacaine, one bupivacaine), but not in any of the seven patients having epidural anesthesia, with incremental dosing probably preventing a major reaction. The proximity of the brachial plexus to large blood vessels, the use of large volumes/doses of local anesthetics, and the use of transarterial techniques probably explains the higher rate of serious complications with upper limb blocks and corroborates the findings of Brown (2) and Auroy (3,4). Most importantly, the conclusion must be that even experts must recognize that accidental intravascular injections are nearly always possible.
More recently, tumescent anesthesia (the extensive subcutaneous infiltration of local anesthetics administered by plastic surgeons for liposuction) has been associated with death secondary to systemic toxicity. Many of these operations were performed in private offices, without an anesthesiologist or any regulatory requirement to report adverse events (even deaths). A typical combination of drugs for tumescent anesthesia is lidocaine 1,000 mg, epinephrine 0.25 to 1.0 mg, and sodium bicarbonate 12.5 mmol/L of crystalloid solution. The injection of doses as great as 55 mg/kg of lidocaine is not uncommon during liposuction. Practitioners claim that most of it is aspirated with the subcutaneous fat that is being removed. However, the dangers of the technique came to prominence with a report (7) to the Chief Medical Examiner of New York City of five deaths after 47,000 procedures, and a survey (8) of U.S. plastic surgeons that estimated that the mortality rate was 1 in 5,224 procedures. As well as excessive plasma lidocaine concentration, death after tumescent anesthesia and liposuction (9) has been attributed to pulmonary embolism, pulmonary edema, epinephrine-induced side effects, excessive sedation, and reduction in venous return due to tight abdominal compression.
In the United Kingdom, death from inadvertent intravascular injection has occurred during continuous epidural
administration using electromechanical infusion devices (10). On two occasions, epidural infusions were connected to IV lines by mistake and, in another, the epidural infusion was connected to the central venous line. The local anesthetic infusion resulted in cardiac arrest and the death of the patient on the operating table. However, these appear not to be isolated events. Between January 2005 and May 2006, the National Patient Safety Agency’s (NPSA) National Reporting and Learning System identified more than 346 incidents involving problems with epidural infusions that resulted in moderate, low, or no harm to patients (10). Reports such as these illustrate how the increased complexity of modern anesthesia, involving multiple monitoring modalities and drug infusions, produces new problems that must be overcome. The risks of regional anesthesia identified by the NPSA concerned the appropriate labeling of solutions and ready discrimination between the electronic pumps and lines used for epidural and other routes of parenteral administration. In considering both these problems, and the complications of tumescent anesthesia described in the previous paragraph, the point must be repeated that operators have a major role in prevention.
administration using electromechanical infusion devices (10). On two occasions, epidural infusions were connected to IV lines by mistake and, in another, the epidural infusion was connected to the central venous line. The local anesthetic infusion resulted in cardiac arrest and the death of the patient on the operating table. However, these appear not to be isolated events. Between January 2005 and May 2006, the National Patient Safety Agency’s (NPSA) National Reporting and Learning System identified more than 346 incidents involving problems with epidural infusions that resulted in moderate, low, or no harm to patients (10). Reports such as these illustrate how the increased complexity of modern anesthesia, involving multiple monitoring modalities and drug infusions, produces new problems that must be overcome. The risks of regional anesthesia identified by the NPSA concerned the appropriate labeling of solutions and ready discrimination between the electronic pumps and lines used for epidural and other routes of parenteral administration. In considering both these problems, and the complications of tumescent anesthesia described in the previous paragraph, the point must be repeated that operators have a major role in prevention.
At the outset, some assumed that the simple substitution of the new drugs (ropivacaine and levobupivacaine) for bupivacaine would eradicate the problem of systemic toxicity. However, overwhelming evidence (as just summarized) from national surveys, FDA and European regulatory bodies, and patient safety groups suggests that local anesthetic systemic toxicity still occurs and remains a source of patient mortality. Part of the problem may be an overly simplistic view of local anesthetic pharmacology, especially among nonanesthesiologists, but another reason is that the nature of regional anesthesia has changed. The increased popularity of major limb blocks, a tendency to inject greater doses of the allegedly “safer” local anesthetics, and wider use of electronic infusion devices have all created a new milieu within which systemic toxicity may occur. Unfortunately, its very nature makes systemic toxicity very difficult to study formally in the clinical setting. To understand the basis of systemic toxicity and develop strategies for its management, most of the studies, in vitro and in vivo, have been in a range of animal models, albeit supplemented with some evidence from human volunteer studies and the clinical arena. Proper understanding of the problem requires that all levels of evidence, from ion channels to man, need to be reviewed, starting with the physicochemical and chiral properties of the drugs themselves.
Table 5-2 Physicochemical characteristics of local anesthetics | ||||||||||||||||||||||||||||||||||||||||||||||||||||||||||||||||||||||||||||||||||||||||||||||||
---|---|---|---|---|---|---|---|---|---|---|---|---|---|---|---|---|---|---|---|---|---|---|---|---|---|---|---|---|---|---|---|---|---|---|---|---|---|---|---|---|---|---|---|---|---|---|---|---|---|---|---|---|---|---|---|---|---|---|---|---|---|---|---|---|---|---|---|---|---|---|---|---|---|---|---|---|---|---|---|---|---|---|---|---|---|---|---|---|---|---|---|---|---|---|---|---|
|
Individual Drug Characteristics
Detailed consideration of the pharmacology of individual agents has been provided in Chapters 2 and 3, with physicochemical drug characteristics (pKa, lipid solubility, protein binding, vasoactivity, and stereoisomerism) to illustrate important determinants of the differences between drugs. Generally, systemic toxicity is regarded, as noted already, as relating primarily to lipid solubility, just as is local anesthetic potency, but a few other factors are important. Vasoactivity (11,12) has some relevance, with most drugs causing a degree of vasodilatation that will increase the rate of absorption. Some, however, such as prilocaine and ropivacaine, are less vasoactive. Prilocaine is also sequestered in lung tissue and is metabolized in the liver more rapidly than other amide drugs, making it a preferred agent for blocks with a relatively high risk of toxicity, the classic example being IV regional anesthesia. The degree of binding to plasma proteins is often taken as an indicator of the risk of toxicity, the erroneous argument being that drugs that are highly protein-bound (such as bupivacaine) are “retained” in the plasma, and do not cross into the tissues to exert a toxic effect. In fact, simply relating the data (Table 5-2) for protein binding to the known clinical risk (see Fig. 4-8) will show that the least protein-bound of the amides (prilocaine) is actually the least toxic, and the most protein-bound (bupivacaine) the most toxic. The final factor to be mentioned comes as consequences of more than 20 years’ of investigation into the basis of bupivacaine cardiotoxicity: namely, extensive consideration of the role of stereoisomerism and drug chirality in its generation.
Chirality, the existence of structural stereoisomers, is a characteristic of many chemicals, and it may influence both the potency and toxicity of local anesthetics (see Table 5-3 for definitions).
One of the drugs introduced as a long-acting alternative to bupivacaine, levobupivacaine, is simply a single isomer derivative of the original drug. They are both potent, lipid-soluble local anesthetics, and have exactly the same physicochemical characteristics; the only difference between them is the number of isomers in solution. Their identical high lipid solubility (partition coefficient 346) and pKa (8.1) imply that the two drugs will cross physiologic membranes equally readily, whether at the cell wall or at intracellular structures such as mitochondria.
The other newer drug, ropivacaine, is also a single S isomer, and comes from the same homologous series as bupivacaine, but the butyl side chain is replaced by a propyl group. This results in a lower partition coefficient (115), which implies that the drug will be less potent as well as less toxic. However, ropivacaine may produce less vasodilatation, and this might compensate for a lower inherent potency by increasing uptake into tissues (14). The underlying principle in the development of both ropivacaine and levobupivacaine was that if a significant difference in cardiotoxicity between the new agents and bupivacaine was coupled with equivalent potency for nerve block, then the gain in therapeutic index warranted their clinical use.
The other newer drug, ropivacaine, is also a single S isomer, and comes from the same homologous series as bupivacaine, but the butyl side chain is replaced by a propyl group. This results in a lower partition coefficient (115), which implies that the drug will be less potent as well as less toxic. However, ropivacaine may produce less vasodilatation, and this might compensate for a lower inherent potency by increasing uptake into tissues (14). The underlying principle in the development of both ropivacaine and levobupivacaine was that if a significant difference in cardiotoxicity between the new agents and bupivacaine was coupled with equivalent potency for nerve block, then the gain in therapeutic index warranted their clinical use.
Table 5-3 Chiral terminology | ||||||||||
---|---|---|---|---|---|---|---|---|---|---|
|
Mechanisms of Toxicity
Early Investigations
Before the public exposure of bupivacaine toxicity in 1979, researchers had already conducted in vitro studies of the dextro (D) and levo (L) isomers of bupivacaine. Both Aberg (15) and Luduena (16), identified (in a variety of small animal studies) a difference in toxicity between enantiomers, showing that levobupivacaine had a 66% to 76% higher median lethal dose (LD50) than D-bupivacaine, and a 41% to 45% higher LD50 than bupivacaine. Studies after Albright’s disclosure sought to determine further the dose and concentration relationships between convulsions and lethality. Intriguingly, a study in mice (17) showed a consistent ratio between the median convulsant (CD50) and lethal doses of local anesthetics. The CD50-to-LD50 ratio was 1.0 for bupivacaine and 1.2 for lidocaine, indicating a very narrow margin between convulsive and cardiotoxic effect, but no obvious difference in the “margin of safety” between these two systemic toxic effects. However, further studies with dog (18,19,20) and sheep (21,22) models in the mid 1980s demonstrated that bupivacaine was associated with the early onset of severe arrhythmias, these often occurring before the onset of convulsions, and that pregnancy increased susceptibility to systemic toxicity (23). Thus, accumulating evidence showed that bupivacaine was much more toxic than previously believed, and that its toxicity was substantially out of proportion to its clinical potency relative to lidocaine. These early studies were the basis for further investigation of the differential effects of local anesthetics on sodium (Na+), potassium (K+), and calcium (Ca2+) channels; mitochondrial function; myocardial electrical conduction; contractility; and the genesis of arrhythmias.
Sodium Channel Block
Local anesthetics preferentially block Na+ channels when they are in the open (conducting) or inactivated (nonconducting) states, as opposed to the resting (nonconducting) state (24). Opportunities for binding to open or inactivated Na+ channels are enhanced by increased frequency of nerve depolarization; this phenomenon is described as phasic or use-dependent block. However, recovery from block during repolarization (the onset of diastole in cardiac muscle) is slow, the dissociation time constant for bupivacaine being some tenfold slower than that for lidocaine. Bupivacaine block of cardiac Na+ channels can be described as being “fast-in, slow-out,” and allowing substantial block to accumulate in the physiologic heart rate range, prolonging conduction, and inducing reentry-type arrhythmias. Metabolic changes, such as hypoxia, acidosis, and hyperkalemia, further enhance systemic toxicity by increasing the proportion of Na+ channels in the inactivated state during diastole.
Cardiac Na+ channels arise from a specific gene, and may have important electrophysiologic differences from Na+ channels in nerve cells. Moreover, the action potential in axons consists of a very brief depolarization due to rapid influx of Na+ ions followed immediately by an equally rapid repolarization due to channel inactivation and termination of Na+ ion flux. In contrast, the action potential of cardiac cells is prolonged, repolarization being delayed by Ca influx during the “plateau” phase, when local anesthetic binding is favored because most Na+ channels are in the inactivated state. Experimentally, Na+ channel block by local anesthetics may be measured in electrophysiologic studies by measuring the maximum upstroke velocity of the action potential (Vmax) and the action potential duration (APD), studies that are often performed in guinea pig papillary muscle. Translated to the clinical EKG, reductions in Vmax correlate with QRS widening, and changes in APD are associated with ventricular arrhythmias related to lengthening of the QT interval.
Data from several studies using the whole-cell voltage clamp technique in isolated cardiac muscle showed that both bupivacaine (25,26) and dextrobupivacaine (27,28) suppressed Vmax more than lidocaine, ropivacaine (25,26), or levobupivacaine (26,27,28) (Table 5-4). Furthermore, recovery from block was faster with levobupivacaine and ropivacaine, implying that systemic toxicity due to these drugs may be easier to overcome. In addition, Vanhoutte et al. (27) and Valenzuela et al. (28) demonstrated, also in isolated guinea pig papillary muscle, that the enantiomers of bupivacaine possess marked stereoselectivity in their effects on the inactivated Na+ channel. The D-enantiomer reduced Vmax, between 65% and 72% more than the L, and shortened the action potential duration more (Table 5-4). Phasic (or frequency-dependent) block may also exhibit stereospecific features (29). Although no differences were seen in Vmax when D-bupivacaine, L-bupivacaine, and ropivacaine were applied to crayfish giant axons stimulated at a frequency of 0.1 Hz, a significant decrease in Vmax occurred (in the order L-bupivacaine >D-bupivacaine >ropivacaine) when the stimulation rate was 5 Hz, suggesting a greater frequency dependent effect of L-bupivacaine.
One reason for the development of cardiac arrhythmias is a concentration and use-dependent slowing of ventricular conduction, progressing to a degree that might allow
electrical reentry. Isolated frozen rabbit hearts, but with a thin layer of surviving epicardial muscle, were stimulated using 256 unipolar minielectrodes, attached to a programmable constant-current stimulator, in the presence of 0.1, 1.0, and 10 μM concentrations of bupivacaine, L-bupivacaine, or ropivacaine (30). Use-dependency was determined by pacing cycle length (PCL), longitudinal ventricular conduction velocity (θL), transverse ventricular conduction velocity (θT), conduction velocities, and the maximal decrease in conduction velocity (Emax) at a PCL of 600 ms. In addition, the ventricular effective refractory period was calculated. Each local anesthetic induced a use-dependent slowing in θL (bupivacaine = L-bupivacaine), and θT (ratio of bupivacaine to L-bupivacaine/ropivacaine of 1:0.74 at 600 ms), and a concentration-dependent prolongation in ventricular effective refractory period (bupivacaine = L-bupivacaine >ropivacaine). The results suggest that equimolar concentrations of ropivacaine are less cardiotoxic than bupivacaine because of less use-dependent conduction block, and that L-bupivacaine cardiotoxicity is intermediate.
electrical reentry. Isolated frozen rabbit hearts, but with a thin layer of surviving epicardial muscle, were stimulated using 256 unipolar minielectrodes, attached to a programmable constant-current stimulator, in the presence of 0.1, 1.0, and 10 μM concentrations of bupivacaine, L-bupivacaine, or ropivacaine (30). Use-dependency was determined by pacing cycle length (PCL), longitudinal ventricular conduction velocity (θL), transverse ventricular conduction velocity (θT), conduction velocities, and the maximal decrease in conduction velocity (Emax) at a PCL of 600 ms. In addition, the ventricular effective refractory period was calculated. Each local anesthetic induced a use-dependent slowing in θL (bupivacaine = L-bupivacaine), and θT (ratio of bupivacaine to L-bupivacaine/ropivacaine of 1:0.74 at 600 ms), and a concentration-dependent prolongation in ventricular effective refractory period (bupivacaine = L-bupivacaine >ropivacaine). The results suggest that equimolar concentrations of ropivacaine are less cardiotoxic than bupivacaine because of less use-dependent conduction block, and that L-bupivacaine cardiotoxicity is intermediate.
Table 5-4 In vitro and isolated organ studies | ||||||||||||||||||||||||||||||||||||||||||||||||||||||||||||||||||||||||||||||||||||||||||||||||||||||||||||||||||||||||||||||||||||||||||||||||||||||||||||||||||
---|---|---|---|---|---|---|---|---|---|---|---|---|---|---|---|---|---|---|---|---|---|---|---|---|---|---|---|---|---|---|---|---|---|---|---|---|---|---|---|---|---|---|---|---|---|---|---|---|---|---|---|---|---|---|---|---|---|---|---|---|---|---|---|---|---|---|---|---|---|---|---|---|---|---|---|---|---|---|---|---|---|---|---|---|---|---|---|---|---|---|---|---|---|---|---|---|---|---|---|---|---|---|---|---|---|---|---|---|---|---|---|---|---|---|---|---|---|---|---|---|---|---|---|---|---|---|---|---|---|---|---|---|---|---|---|---|---|---|---|---|---|---|---|---|---|---|---|---|---|---|---|---|---|---|---|---|---|---|---|---|---|---|
|
Potassium Channel Block
Potassium channels (all of which may interact with local anesthetics) are a relatively heterogeneous group compared to Na+ channels. Three basic forms exist, with the following nomenclature:
Six transmembrane segments, single-pore, voltage-gated channel (31,32,33). Examples include Kv1 to Kv9, and human ether-a-go-go-related potassium (HERG) channels (34,35).
Two transmembrane segments, single-pore, inward rectifier channel. Examples include Kir 1,1, 1.2, 3.1, 6.2, and others (36,37), which are important in setting the resting membrane potential.
During the cardiac action potential, K+ channels are responsible for repolarization and stabilization of the cell membrane resting potential. Potassium channel block contributes to the systemic toxicity of local anesthetics by lengthening the cardiac action potential, predisposing the heart to ventricular arrhythmias such as torsade de pointes. Evidence of K+ ion involvement in systemic toxicity and a propensity for cardiac arrhythmias is suggested by prolongation of the Q-Tc interval on a 12-lead EKG (40). Further evidence for the effects of ropivacaine, L-bupivacaine, and D-bupivacaine was obtained by studies on cloned human cardiac delayed- rectifier K+ channels expressed in a mouse fibroblast cell line, using a patch clamp technique. Apparent dissociation constant (KD) values of 27.3 μM and 4.1 μM were calculated for L-bupivacaine and D-bupivacaine, respectively, indicating that the latter has sevenfold greater affinity for the K+ channel (31). Using the same model, the same research group found that ropivacaine had an apparent KD of 80 μM (41), suggesting that the relative potency of local anesthetic K+ channel block is in the order bupivacaine >L-bupivacaine >ropivacaine. In contrast, the optical isomers of mepivacaine showed no stereospecific effect (42).
Bupivacaine, L-bupivacaine, and ropivacaine were also shown to block both the open and inactivated states of HERG channels in a time- and concentration-dependent manner. However, the evidence for stereoselective block is conflicting. Gonzalez et al. (33) found that L-bupivacaine was twice as potent as D-bupivacaine in blocking HERG channels, whereas Friederich et al. (34) showed no stereospecific effect at HERG and HERG/MiRP1 channels. Studies on tandem-pore domain K+ channels (2P K+) also produced some controversy (39). These channels are widely expressed in the CNS, and are involved in the control of the resting membrane potential and the firing pattern of excitable cells. Thus, their inhibition results in membrane depolarization, increasing the affinity of both open and inactivated Na+ channels for local anesthetics. TASK-2, but not TASK-1, K+ channels showed agent-specific, dose-dependent, and stereoselective responses (43), with half maximal inhibitory concentration (IC50) values of 17 μM and 43 μM for D-bupivacaine and L-bupivacaine, and 85 μM and 236 μM for D-ropivacaine and ropivacaine, respectively (Table 5-4).
Calcium Channel Block
Calcium ions stimulate myocardial contractility by passing through long-lasting (L)-type Ca2+ channels, binding to ryanodine receptors in the sarcoplasmic reticulum, and triggering Ca-induced Ca release (44). Relaxation of the myocardium is an energy-dependent process, and is reliant on the removal of Ca from troponin binding sites and active return of Ca to the sarcoplasmic reticulum by an adenosine triphosphate (ATP)-dependent regulatory protein called SERCA2.
Rossner (45) showed that bupivacaine inhibited cardiac Ca2+ channels tonically in a concentration-dependent manner. Zapata-Sudo et al. (46) subsequently investigated the effect of D-bupivacaine and L-bupivacaine on L-type calcium channels in isolated perfused rat ventricular myocytes. At a concentration of 10 μM, significant differences occurred between the isomers (D >L) in the incidence of ventricular arrhythmias, and lengthening of the PR and QRS intervals of the EKG. In addition to these global stereospecific differences, specific inhibitory effects on Ca2+ channels were investigated using the whole-cell clamp technique. Maximal reduction of inward Ca currents (ICa) was 57% for D-bupivacaine 20 μM and 51% for L-bupivacaine 10 μM, indicating that differences between the isomers could be attributed to stereoscopic effects at Na+ and K+ channels, but not Ca channels.
Thus, the propensity of local anesthetics to produce systemic toxicity, in the forms of myocardial depression and poor response to traditional resuscitation, may be only partially explained by stereoselective ion channel block; local anesthetics also have intracellular actions linked to the bioenergetics of the mitochondria (47,48,49).
Effects on Mitochondrial Function
Much recent work on local anesthetic toxicity relates to effects on mitochondrial function, an important area, because 70% of myocardial energy needs are supplied by the oxidation of fatty acids. Discussion of the cellular mechanisms of actions of local anesthetics requires first a description of the pathways involved in fatty acid breakdown.
Long-chain fatty acids react with coenzyme A (CoA) in an ATP-dependent process catalyzed by long-chain acyl-CoA synthetase (LCAS) located in the outer mitochondrial membrane (Fig. 5-2). To cross the inner mitochondrial membrane, Acyl-CoA is transformed into acylcarnitine by carnitine palmitoyltransferase I (CPT I), located in the outer membrane. After crossing the inner membrane, acylcarnitine is then regenerated to acyl-CoA by carnitine palmitoyltransferase II (CPT II) within the matrix compartment, and acyl-CoA is metabolized to H2O
and CO2 in the tricarboxylic acid cycle. In tissues, almost 90% of ATP is formed by a mitochondrial process termed oxidative phosphorylation. Located within the inner mitochondrial membrane is a chain of “respiratory” enzymes and coenzymes that transport hydrogen or electrons, developing enough energy to transport protons from the mitochondrial inner compartment or matrix into the intermembrane space.
and CO2 in the tricarboxylic acid cycle. In tissues, almost 90% of ATP is formed by a mitochondrial process termed oxidative phosphorylation. Located within the inner mitochondrial membrane is a chain of “respiratory” enzymes and coenzymes that transport hydrogen or electrons, developing enough energy to transport protons from the mitochondrial inner compartment or matrix into the intermembrane space.
![]() Figure 5-2. An ATP-dependent process catalyzed by long-chain acyl-CoA synthetase (LCAS) located in the outer mitochondrial membrane. |
It has been known for several years that lipophilic local anesthetics interfere with mitochondrial energy-linked processes by uncoupling oxidative phosphorylation on complex I of the intracellular mitochondrial respiratory chain (50,51) and inhibiting respiratory chain enzymes (52,53). In addition, local anesthetics inhibit state 3, active respiration (oxygen consumed in converting ADP to ATP), and state 4 respiration (oxygen consumed in the resting state to maintain the chemiosmotic gradient) (54). Furthermore, less disturbance of mitochondrial energy metabolism by ropivacaine (55), hence less reduction of cAMP, indicated that this effect correlates with lipid solubility (56), but not stereoisomerism (57).
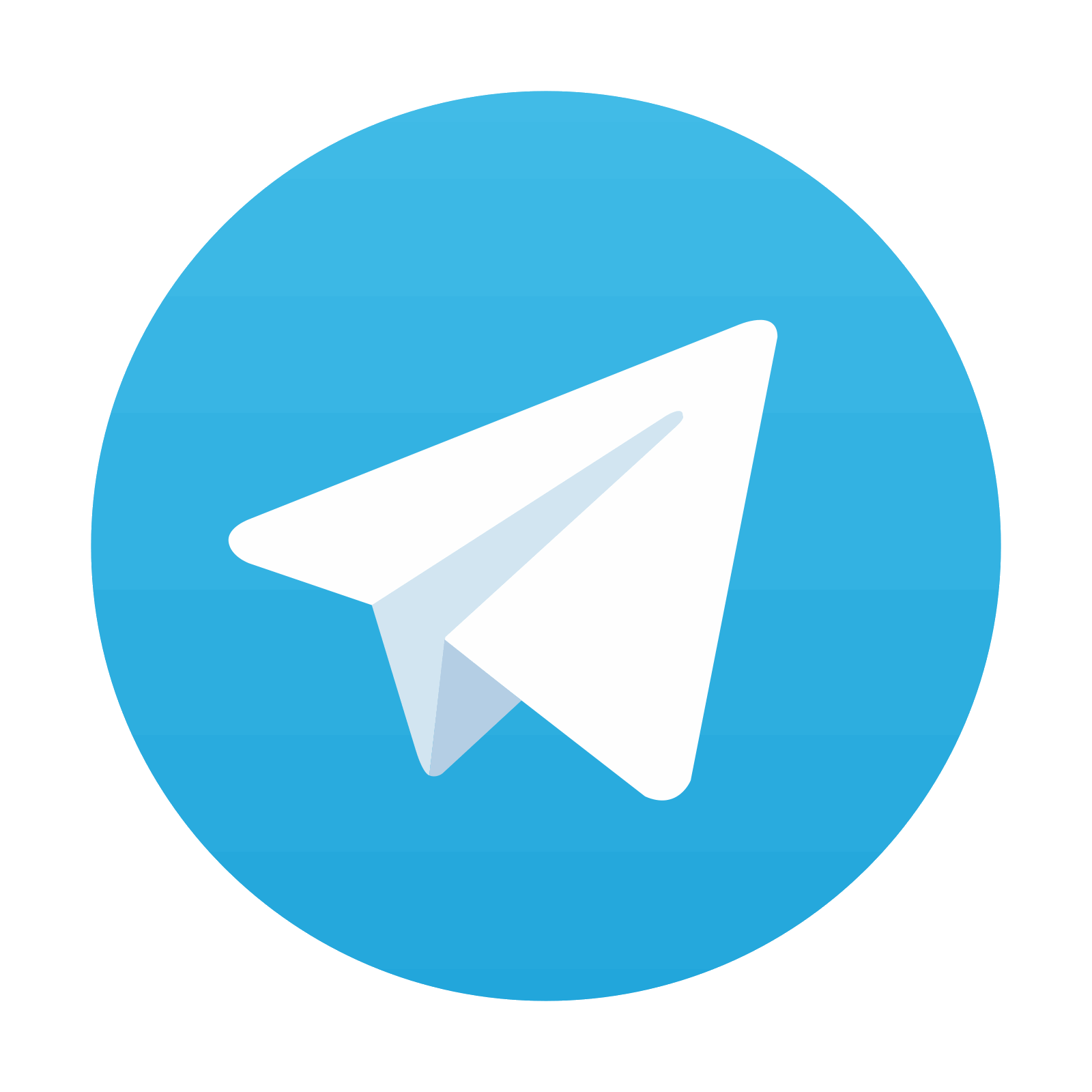
Stay updated, free articles. Join our Telegram channel
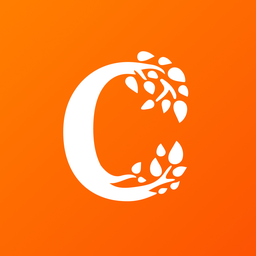
Full access? Get Clinical Tree
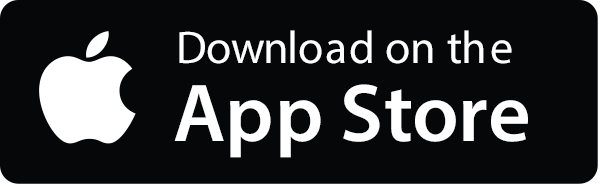
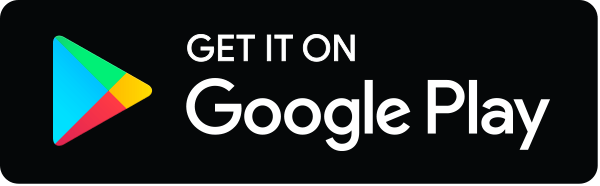