Local Anesthetic Pharmacology
Francis V. Salinas
KEY POINTS
1. Local anesthetics must penetrate through several extraneural connective tissue layers to reach the axonal membrane.
2. Local anesthetics provide anesthesia and analgesia by blocking the transmission of painful sensory stimuli along nerve fibers.
3. The action potential is mediated by inward sodium currents and outward potassium currents through their respective voltage-gated ion channels.
4. Local anesthetics bind to the voltage-gated sodium channels, interrupting the rapid inward current of sodium ions and generation of the action potential; thereby inhibiting the transmission of neural impulse.
5. Aminoamide local anesthetics are metabolized in the liver, and aminoester local anesthetics are metabolized by plasma esterase.
6. Local anesthetic potency is related to the physiochemical properties of the specific local anesthetic agent. Increasing lipid solubility increases potency and duration of action.
7. The analgesic efficacy and/or duration of action of local anesthetics may be augmented by the addition of adjuvant agents.
8. Total dose, site of administration, and patient-specific factors (age, cardiovascular and hepatic function, and plasma protein binding) influence subsequent plasma levels and the potential for systemic toxicity.
LOCAL ANESTHETICS ARE A CLASS OF DRUGS that transiently and reversibly inhibit the conduction of sensory, motor, and autonomic neural impulses. They are the primary medications used to provide perioperative regional anesthesia and analgesia. This chapter presents the mechanism of action of local anesthetics, the physiochemical properties that determine their clinical pharmacology, common clinical applications, and potential for toxicity. Karl Koller reported the first clinical use of local anesthetics (cocaine) in 1884 to provide topical anesthesia for ophthalmologic surgery.
I. Clinical anatomy of nerve conduction
A. Anatomy of nerves. The neuron is the basic functional unit responsible for the conduction of neural impulses. It consists of a cell body which is attached to several branching processes (dendrites) and a single axon that transmit neural impulses toward and away from the cell body, respectively (Fig. 5.1). Axons are cylinders of axoplasm encased within a phospholipid bilayer cell membrane that is embedded with various membrane-spanning channel proteins. The most
important of these protein channels include voltage-gated sodium and potassium channels and the sodium-potassium pump. Schwann cells are closely associated with neurons and function to support, insulate, and nourish axons. The cell membrane of Schwann cells (neurolemma) immediately surround axons. A peripheral nerve fiber is composed of an axon, its associated neurolemma, and the surrounding endoneural connective tissue.
important of these protein channels include voltage-gated sodium and potassium channels and the sodium-potassium pump. Schwann cells are closely associated with neurons and function to support, insulate, and nourish axons. The cell membrane of Schwann cells (neurolemma) immediately surround axons. A peripheral nerve fiber is composed of an axon, its associated neurolemma, and the surrounding endoneural connective tissue.
B. Peripheral nerve classification. Peripheral nerves contain both afferent and efferent nerve fibers that are classified as either myelinated or nonmyelinated (Fig. 5.1). A myelinated nerve fiber is segmentally encased by a continuous series of Schwann cells that produce myelin. Multiple concentric lipid layers of myelin form a specialized neurolemma (myelin sheath) around a single axon. Specific regions (termed nodes of Ranvier), where the voltage-gated sodium channels [VGNa] are concentrated along the axons of myelinated nerve fibers periodically interrupt the myelin sheath. Along myelinated axons, Na+ conductance is restricted to the nodes of Ranvier. This allows action potential propagation to jump from one node to another via saltatory conduction, which significantly enhances the speed of signal transmission (Table 5.1). In contrast, nonmyelinated nerve fibers consist of multiple axons that are simultaneously encased within the neurolemma of a single nonmyelin producing Schwann cell. VGNa are uniformly distributed along the entire axon of nonmyelinated nerve fibers.
C. Peripheral nerve fiber microanatomy. Peripheral nerve fibers are organized within four layers of connective tissue (Fig. 5.2).
1. Individual nerve fibers are encased within endoneurium, which contains loose connective tissue that consists of Schwann cells, fibroblasts, and capillaries.
2. A dense layer of collagenous connective tissue, the perineurium, encloses bundles of nerve fibers into a fascicle. All but the smallest peripheral nerves are arranged within fascicles. The perineurium functionally provides an effective barrier against penetration of the nerve fibers by foreign substances.
TABLE 5.1 Classification of peripheral nerve fibers | ||||||||||||||||||||||||||||||||||||||||||||||||||||||||
---|---|---|---|---|---|---|---|---|---|---|---|---|---|---|---|---|---|---|---|---|---|---|---|---|---|---|---|---|---|---|---|---|---|---|---|---|---|---|---|---|---|---|---|---|---|---|---|---|---|---|---|---|---|---|---|---|
|
3. The epineurium is also a dense connective tissue layer that surrounds and encases bundles of fascicles together into a cylindrical sheath structurally resembling a coaxial cable.
4. An additional connective tissue layer, the paraneurium (paraneural sheath), further encases peripheral nerves (1,2). Together, these tissue layers not only offer protection to peripheral nerves but also present a significant barrier to passive diffusion of local anesthetics toward the axonal cell membrane.
II. Electrophysiology of neural conduction and voltage-gated sodium channels
A. Resting membrane potential (Fig. 5.3A). Neurons maintain a resting membrane potential of approximately -60 to -70 mV. The Na+-K+ (potassium) pump actively cotransports three Na+ ions out of the cell for every two K+ ions into the cell. The resulting ionic disequilibrium favors the passive movement of Na+ ions into the cell and K+ ions out of the cell. However, despite the concentration gradient for both ions, the resting cell membrane is selectively more permeable to K+ ions. This facilitates a net passive efflux of K+ ions out of the cell and leaves a relative
net excess of negatively charged ions (polarized) within the axoplasm. This creates the resting electrochemical concentration gradient across the semipermeable cell membrane.
net excess of negatively charged ions (polarized) within the axoplasm. This creates the resting electrochemical concentration gradient across the semipermeable cell membrane.
B. The action potential (Fig. 5.3B). Neural impulses are conducted along the axonal cell membrane as action potentials, which are transient membrane depolarizations initiated by various mechanical, chemical, or thermal stimuli. Depolarization is mediated primarily via
rapid intracellular influx of Na+ ions flowing down its electrochemical gradient through VGNa. The VGNa spans the axonal membrane and consists of an α-subunit and one or two varying auxiliary β-subunits. At the resting membrane potential, the VGNa is in a resting (closed) conformation. Upon an initial depolarization, a conformational change results in activation (opening) of the VGNa, inducing a sudden increase in Na+ ion permeability. The resultant rapid inward Na+ current activates and opens additional VGNa. This further accelerates depolarization until a threshold membrane potential is reached, triggering an action potential. During the depolarization phase, the inward Na+ current flows into the axoplasm and spreads to the adjacent (inactive) cell membrane, resulting in a wave of sequential depolarization (and the action potential) propagating along the axon. Although the wave of depolarization spreads from the initial area of excitation in both directions, the just activated membrane behind the impulse is temporarily refractory (owing to inactivation of VGNa) to subsequent depolarization. Thus, the propagation of the impulse is unidirectional.
rapid intracellular influx of Na+ ions flowing down its electrochemical gradient through VGNa. The VGNa spans the axonal membrane and consists of an α-subunit and one or two varying auxiliary β-subunits. At the resting membrane potential, the VGNa is in a resting (closed) conformation. Upon an initial depolarization, a conformational change results in activation (opening) of the VGNa, inducing a sudden increase in Na+ ion permeability. The resultant rapid inward Na+ current activates and opens additional VGNa. This further accelerates depolarization until a threshold membrane potential is reached, triggering an action potential. During the depolarization phase, the inward Na+ current flows into the axoplasm and spreads to the adjacent (inactive) cell membrane, resulting in a wave of sequential depolarization (and the action potential) propagating along the axon. Although the wave of depolarization spreads from the initial area of excitation in both directions, the just activated membrane behind the impulse is temporarily refractory (owing to inactivation of VGNa) to subsequent depolarization. Thus, the propagation of the impulse is unidirectional.
C. Repolarization (Fig. 5.3C and D). The activated VGNa is inactivated within milliseconds by an additional conformational change. This rapid inactivation process is required for repetitive firing of action potentials in neural circuits and for control of excitability in neurons. Repolarization occurs because of a combination of a progressive decrease in the driving force for the inward Na+ current and inactivation of VGNa. In addition, membrane depolarization simultaneously activates voltage-gated K+ channels. This leads to an outward positive current of K+ ions, which in conjunction with VGNa inactivation, eventually returns the axonal membrane to or just beyond (hyperpolarization) its resting membrane potential.
D. In summary, inward positive currents, mediated by Na+ ions, depolarize the membrane, and in contrast, outward positive currents, mediated by K+ ions, repolarize the membrane.
III. Voltage-gated sodium channels and interactions with local anesthetics. The prototypical local anesthetic structure consists of a hydrophobic group (typically a lipid-soluble aromatic ring) connected to a tertiary amine group by either an amide or ester linkage (Fig. 5.4). Because the tertiary amine group can accept a proton to become a positively charged quaternary amine, local anesthetics exist in equilibrium as a neutral (base, more hydrophobic form) or the protonated (charged, more hydrophilic form). Local anesthetics must first cross the axonal membrane to reach the local anesthetic binding site. The neutral form more readily crosses the axonal membrane. The charged form is responsible for interacting with the local anesthetic binding site (Fig. 5.4).
Local anesthetics act at the axonal membrane by binding to a specific region within the α-subunit of the VGNa (3). This prevents VGNa activation, thus inhibiting the inward Na+ current that mediates membrane depolarization. The binding site may be approached from two pathways: from the intracellular aspect of the channel pore (hydrophilic pathway) or laterally from within the lipid membrane (hydrophobic pathway) (Fig. 5.4). As the amount of administered local anesthetic increases, an increasing percentage of VGNa can bind to local anesthetics, further inhibiting the inward Na+ current. Subsequently, the rate of depolarization (in response to stimulation) is attenuated, inhibiting the achievement of the threshold membrane potential. Consequently, achievement of an action potential becomes increasingly difficult. With enough local anesthetic-bound VGNa, an action potential can no longer be generated and impulse propagation is blocked.
A. Local anesthetics bind more avidly to VGNa in the activated (open) and inactivated (closed) conformations. The difference in binding affinity is attributable to the difference in the availability of the two pathways for local anesthetic to reach the binding site. Local anesthetics produce a concentration-dependent decrease in inward Na+ current characterized as tonic blockade, representing a decrease in the number of open confirmation VGNa.
B. With repeated depolarization, a greater number of VGNa are in either the activated or inactivated conformations. Therefore, they can be bound at a lower local anesthetic concentration. Additionally, the dissociation rate of local anesthetics from their binding site is slower than the rate of transition from the inactivated to the resting conformation. Thus, repeated stimulation results in accumulation of local anesthetic-bound VGNa characterized as frequency-dependent blockade.
IV. Mechanisms of nerve block
A. Local anesthetic concentration and volume. The quality of nerve block is determined not only by the intrinsic potency but also by the concentration and volume of the administered local anesthetic. The potency of a local anesthetic can be expressed as the minimum effective concentration required to establish complete conduction block. The volume of local anesthetic is also important, because a sufficient length of axon or successive nodes of Ranvier must be blocked to inhibit regeneration of the neural impulse. This is caused by the phenomenon of decremental conduction. Membrane depolarization passively decays with distance away from the front of the action potential to the point that impulse propagation stops when depolarization falls below the threshold for VGNa activation. If less than a critical length of axon is blocked, the action potential may still be regenerated in the proximal neural membrane segment or node of Ranvier when the decaying depolarization is still above the threshold potential.
B. Inherent nerve susceptibility to local anesthetic blockade. Different types of nerve fibers demonstrate varying minimal blocking concentrations and local anesthetic susceptibilities (Table 5.1). Clinically, there is a predictable progression of sensory and motor function blockade, starting first with loss of temperature sensation, followed by proprioception, motor function, sharp pain, and light touch. Termed differential block, this progression was initially attributed to differences in axon diameter, with smaller fibers inherently more susceptible to conduction blockade compared with that of larger fibers. However, small myelinated fibers (Aγ and Aδ) are the most susceptible to conduction blockade. Next in order of block susceptibility are large myelinated fibers (Aα and Aβ), and the least susceptible are small, nonmyelinated C fibers.
CLINICAL PEARL
The afferent fibers that conduct well-localized sharp (surgical) pain and cold temperature sensation share similar conduction velocity and local anesthetic susceptibility. Thus, loss of cold temperature sensation provides a qualitative assessment of the onset and distribution of sensory block.
C. Anatomic mechanism. For local anesthetics to bind VGNa, they must initially reach the axonal membrane. Thus, local anesthetics must penetrate through variable amounts of perineural tissue and still maintain a sufficient concentration gradient to diffuse through the phospholipid bilayer.
1. Peripheral nerve block. Only a small fraction (1% to 2%) of local anesthetic reaches the neural membrane even when deposited near peripheral nerves. Peripheral nerves that have been desheathed in vitro require about a hundredfold lower local anesthetic concentration than peripheral nerves in vivo. Within peripheral nerves, longitudinal and radial diffusion of local anesthetic will produce varying drug concentrations along and within the nerve during the onset and recovery from clinical block. When local anesthetics are deposited around a peripheral nerve, diffusion progresses from the outer surface (mantle) toward the center (core) along a concentration gradient. Consequently, the nerve fibers arranged in the mantle of mixed peripheral nerves are blocked initially. These outer nerve fibers are typically distributed to more proximal anatomic structures, whereas core fibers innervate more distal structures. This topographic arrangement explains the initial development of proximal, followed by distal anesthesia, because local anesthetic diffuses to the more centrally located core nerve fibers. In summary, the sequence of onset and recovery from peripheral nerve block depends on a combination of the topographic arrangement of the nerve fibers within a mixed peripheral nerve and their inherent susceptibility to local anesthetic blockade.
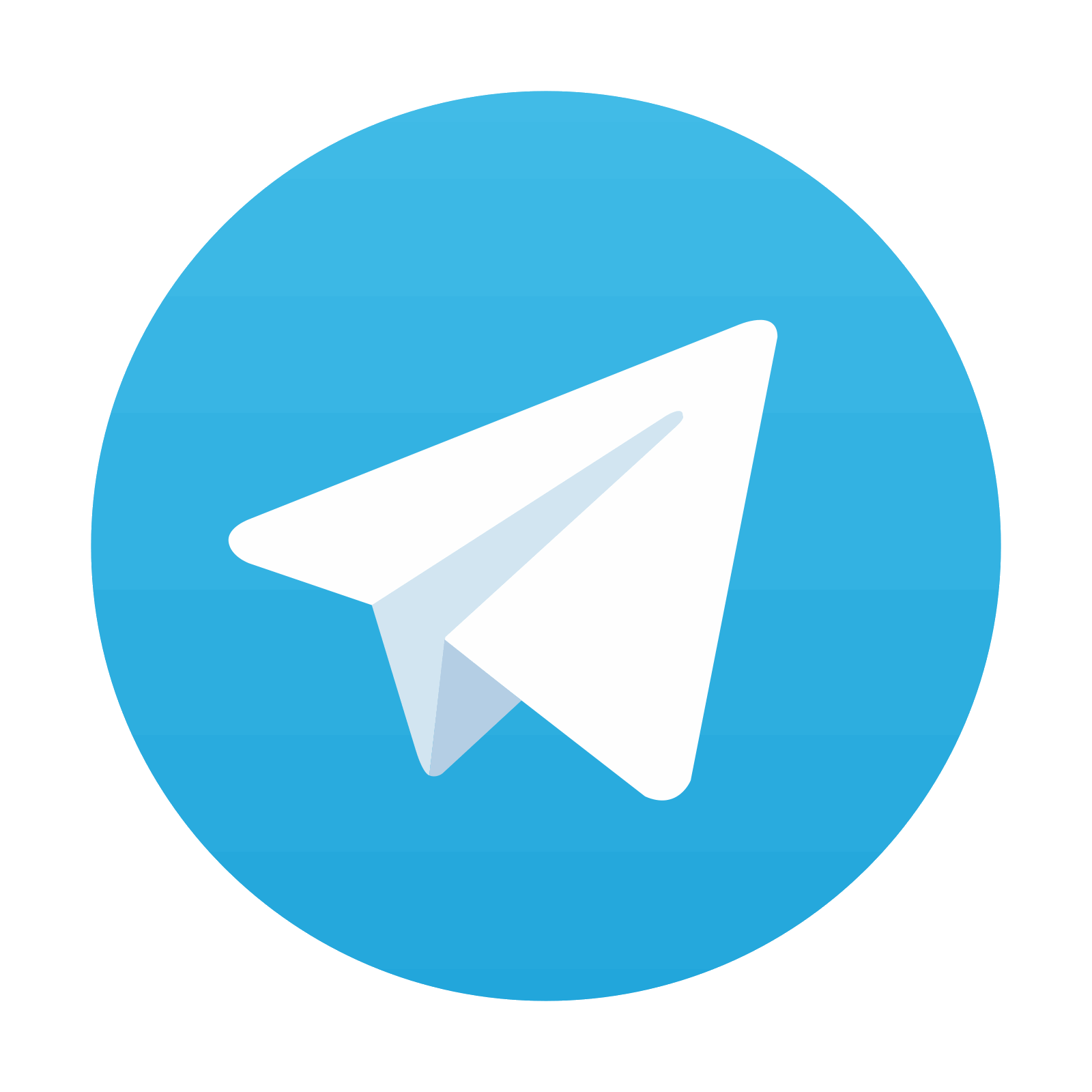
Stay updated, free articles. Join our Telegram channel
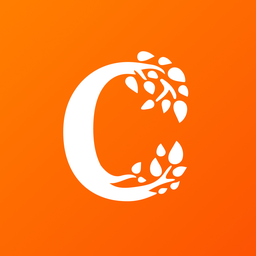
Full access? Get Clinical Tree
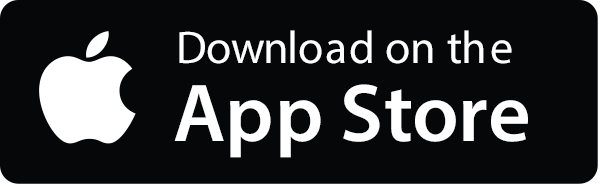
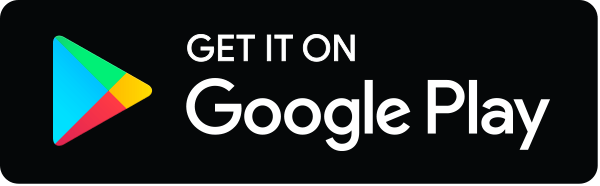