Modality
Age affected
Factors
Manifestation
Adaptive strategy
MN, UN SSEPs
Birth—2 years
Central myelination
Broad, low-amplitude delayed cortical potentials
Decrease stimulus rate; increase pulse length
PTN SSEPs
Birth—4–6 years
Dorsal column myelination
As earlier
As earlier
TcMEPs
Birth—2 years
a
Exquisite sensitivity to volatile agents
Temporal facilitation techniques, high frequency stimulation for CN, UE
D-wave
Birth—2 years
CST myelination
Impossible to record
None known; rely on TcMEPs
EMG
Birth—2 years
b
Low signal amplitude, short duration potentials
Avoid surface pads for intraoperative recording, needle electrodes
BAERs
Birth—1 year
Central myelination
Sensitivity of wave V to volatile agents
TIVA technique
BCR
All
Polysynaptic reflex
Sensitivity to volatile agents
TIVA technique, pulse-train stimulation—double tap or double train with long ITI
Anesthetic Management of Pediatric Surgical Procedures That Require Intraoperative Neurophysiological Monitoring
The anesthesiologist must balance the condition of the patient , the surgical procedure, and the monitoring modalities to be used when selecting an anesthetic technique. Rare metabolic diseases such as mitochondrial myopathies may limit the choice of anesthetic agents that can be safely used. In these cases, the anesthesia team, surgeon, and neurophysiology team must formulate a plan that will provide as much information as possible to guide the procedure while protecting the patient from exposure to potentially hazardous anesthetic agents.
TIVA is the preferred anesthetic technique if TcMEPs are to be elicted. If TIVA is not a feasible option, 0.5 MAC of inhalation agent (desflurane) supplemented with remifentanil can be used in the absence of significant neurological compromise. Higher stimulation intensities may be required to elicit TcMEPs due to volatile agent effects at the level of the alpha motor neurons (αMNs) [1]. Increased stimulus intensities have attendant risks of excessive patient movement and increased risk of bite injuries such as tongue lacerations. The use of volatile agents is also associated with an increased rate of false-positive alerts compared with TIVA [2].
It is generally accepted that healthy young adults, with no pathology involving the pathways involved in IONM, can be anesthetized with either a TIVA or 0.5 MAC desflurane-based anesthetic during monitored (IONM) procedures. This is not the case for young children. The presence of low residual concentrations of sevoflurane, following an inhalation induction, may significantly impair the generation of TcMEPs in the lower extremities. Currently, unpublished data from the author’s institution indicates that volatile agent added over a TIVA, as is sometimes done to suppress patient movement, can dramatically reduce TcMEP amplitudes in the lower extremity muscles of young healthy adolescents. TIVA , with propofol and remifentanil, is the recommended primary anesthetic regimen when IONM is required in pediatric patients. The addition of ketamine (5–20 μg/kg/min) to the anesthetic may help provide improved hemodynamic stability, but care must be taken to terminate a ketamine infusion in a timely manner due to the pharmacokinetic properties of ketamine. Ketamine, propofol, and isoflurane have been shown to cause neurodegeneration in the brains of subhuman primates when used in high doses over a period of time [3]; however, the effects in humans are an area of controversy as these agents may protect from damage due to nociceptive inputs [4]. Some propofol sparing may also be achieved by using dexmedetomidine , but dexmedetomidine must be used judiciously as blood levels greater than 0.6 ng/mL depress the MEPs [1]. A low-dose infusion (0.2 μg/kg/h) without a loading bolus keeps the blood levels below the threshold. If electromyography (EMG) or TcMEPs are used as one of the monitoring modalities, the neuromuscular blocking agents (NMBs) should be avoided if possible. If used for intubation, the use of short-acting agents is preferred as baseline potentials may be acquired within 10–20 min of intubation in some cases. NMBs should be completely avoided, or completely reversed if cranial nerve EMG and/or cranial nerve TcMEPs are to be acquired in young children.
Concern about the ability to assess anesthetic depth with TIVA is cited as a primary reason for not using this technique. Recently published data define the EEG patterns associated with the initial loss of consciousness and maintenance of such loss in healthy volunteers receiving propofol infusions [5, 6], sevoflurane [7], and dexmedetomidine [8]. EEG patterns associated with surgical anesthesia conditions in infants from age 0 to 6 months using sevoflurane have also been published [9]. Similar patterns are also found when using fast-Fourier analysis (FFA ) of frontal montage EEG in patients in the same age groups receiving TIVA anesthetics (Fig. 43.1a–d). Although EEG data can be informative regarding cortical activity, it is not completely reliable as a predictor of movement. The successful use of TIVA relies on the suppression of nociceptive stimuli with sufficient narcotic dosing, as propofol has limited ability to prevent movement in dose ranges compatible with eliciting reliable TcMEPs in the lower extremities of young children. Using a combination of bilateral frontal montage EEG with fast Fourier transform, and EMG, it is possible to monitor both the cortical response to anesthetic dosing and EMG signs of incipient movement. Spontaneous EMG used to monitor the facial and lower cranial nerves has been found to be predictive of incipient patient movement during emergence from anesthesia [10, 11]. We have also found that spontaneous EMG activity in the small muscles of the hands can signify incipient movement as well.
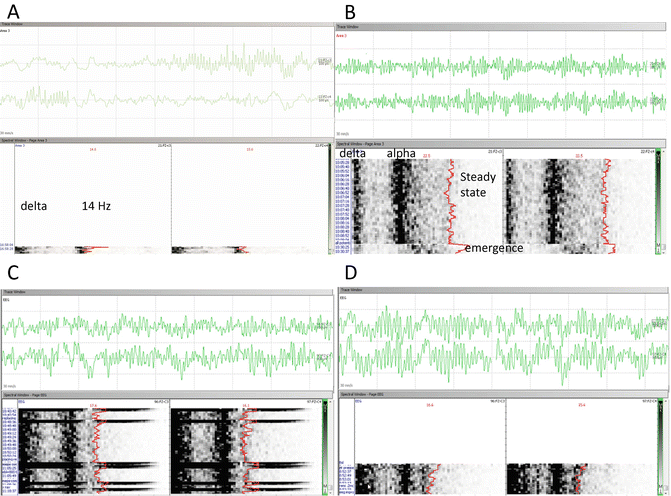
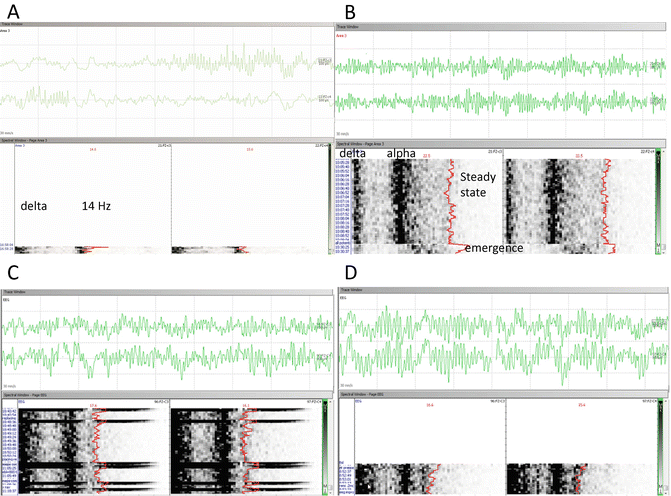
Fig. 43.1
Two-montage frontal EEG/DSA during TIVA anesthesia in children of different ages. (a–d) Real-time raw EEG and time-stacked density spectral array (DSA) acquired during TIVA anesthetics for children of ages 4 months, 14 months, 5 years, and 15 years, respectively. (a) At 4 months of age, the presence of a high-power delta band is associated with a surgical plane of anesthesia. There is also a 14-Hz beta frequency band present in this sample. (b) Dense delta and alpha bands are associated with a surgical plane of anesthesia at this age. The raw EEG from the 14-month-old child was recorded during emergence; the DSA shows a transition from a surgical plane (steady state) to a pattern suggestive of emergence. The EEG and DSA patterns for the 5-year-old child (c) and the 15-year-old child (d) are very similar
Monitoring Modalities and Development
Somatosensory Evoked Potentials
Somatosensory evoked potentials (SSEPs) constitute a set of signals generated by action potentials of nuclei in the CNS and primary sensory cortical neurons in response to stimulation of peripheral nerves; most commonly the median or ulnar nerves for the upper extremities and the posterior tibial nerves for the lower extremities . These signals travel ipsilaterally from the site of the initial peripheral nerve stimulus and then ascend via the dorsal columns, conveying vibration and proprioceptive sensation to either the cuneate (upper extremities) or gracile (lower extremities and trunk) nuclei in the medulla. From there, the response crosses over to the contralateral side in the arcuate fibers and travels to the ventral-posterior lateral tiers of the thalamus via the medial lemniscus. Then the response travels to the cortex through the posterior limb of the internal capsule. Therefore, monitoring SSEPs provides information concerning the integrity of the vibration and proprioceptive sensory tract from the periphery to the primary sensory cortex [12–14]. The basics of SSEPS are covered in more detail in Chap. 1.
The challenge posed by pediatric patients, especially the very young, is that the different parts of the pathways involved in the formation of SSEPs mature at different rates. Myelination of the central pathways is generally complete by 2 years of age, but the myelination of the dorsal columns is not complete until about 8 years of age [15]. The medial lemniscus is usually fully myelinated by 12 months and the thalamocortical projections by 12–18 months of age [16]. As a consequence of the delayed myelination of the dorsal columns, there tends to be greater dispersion of signals traveling up the dorsal columns of a 2-year-old child than an adult, thus resulting in a lower likelihood of generating a useable PTN cortical SSEP in the 2-year-old child. Failure to obtain cortical PTN SSEPs in children younger than 2 years is not uncommon; the rate of failure increases in the face of pathology within the spinal cord and with anesthesia/sedation [17]. We have found that TcMEPs are obtained more frequently than PTN SSEPs when monitoring thoracic and lumbar spinal cord function in children younger than 6 years of age, when using a TIVA technique [18].
The median nerve peripheral responses and cervical potentials have very short latencies in infants and children younger than age 2. Although conduction velocities in the peripheral nerves do not reach adult values until 5 years of age [19], due to the shorter distance between stimulus and recording electrodes, the latencies are much shorter than in an adult. Once the signals reach the medullary nuclei (gracile and cuneate), slower synaptic transmission and slower central conduction times, due to incomplete myelination, result in prolonged peripheral to cortical interpeak latencies and near-adult-value cortical latencies.
Infant cortical SSEPs and cortically dependent neurophysiological signals will, in general, be more sensitive to anesthetic effects than will cortical SSEPs in a school-age child or an adolescent [20]. Recording cortical potentials in this group of children is challenging and is very dependent on the anesthesia team providing a permissive anesthetic and managing it carefully.
When attempting to record SSEPS from an infant or child under 2, the technologist can increase the chance of recording potentials by reducing the stimulus rate and increasing the stimulus pulse width compared to the rate and pulse widths typically used to record SSEPs in adults. Additionally, the waveforms will have altered morphologies; the cortical potentials will have lower amplitudes and longer durations due to the “smearing” effects of conduction velocity dispersion. For many clinical applications, cortical potentials are not essential. For example, when monitoring a tethered cord release, high-quality cervical potentials recorded by using an Fz-C5s montage will provide the essential information concerning the integrity of the sacral roots that comprise the PTN (L4–S3). It is often possible to obtain adequate cervical PTN potentials in the same patient as poorly reproducible PTN cortical potentials ; failure to include the Fz-C5s montage due to “insufficient” channels may obviate any chance of monitoring PTN potentials in young children.
There is one important technical concern when performing SSEPs in infants under 18 months of age, and that relates to the fontanelles . The anterior and posterior fontanelles are midline openings in the skull that, in conjunction with open sutures, permit rapid brain growth during infancy. The anterior fontanelle may initially enlarge after birth but then decrease in size after 6 months of age. It is typically closed to palpation between 9 and 18 months of age. The anterior fontanelle should be located in young infants as it is proximate to the Fz site. Needle placement at or close to the anterior fontanelle should be avoided. Some infants have a palpable wide metopic suture in the frontal midline. If found, needles should be placed off the midline. The posterior fontanelle is typically closed to palpation by 4 months of age.
Transcranial Motor Evoked Potentials
Within 10 years of the adoption of SSEPs as a means to monitor scoliosis surgery, it was noted that postoperative deficits could occur despite unchanged SSEPs [21]. In response, MEPs were introduced, allowing assessment of the integrity of the large diameter corticospinal tract (CST) axons and the αMNs.
The basic neurophysiology of motor evoked potentials is discussed in Chap. 2, “Transcranial Motor Evoked Potentials.” The introduction of MEPs into pediatric clinical practice was motivated by the observation that intra- and postoperative motor deficits can occur without a sensory deficit as monitored by SSEPs. Therefore, it is necessary to monitor the functional integrity of the motor tracts of the spinal cord apart from the sensory tracts. MEPs serve this purpose. MEPs are usually elicited by applying a high voltage, short duration stimulus to the scalp overlying the primary motor cortex. This electrical stimulus depolarizes the axons of the pyramidal neurons (D waves) and the interneurons (I waves) [22]. The D and I waves are conducted along the CST to activate the spinal αMNs [23]. The MEP, recorded from a pair of needle electrodes placed in the muscle groups of interest, represents the compound muscle action potential (CMAP ) initiated by the D and I waves. The amplitude, latency, and morphology of the CMAP are the criteria to assess the integrity of the motor pathways. Criteria have been developed for issuing an alert to the surgical team due to changes in the MEPs [24–26]. There are no published large series examining the application of these criteria for alerting the surgical team to changes in children under age 6; however, clinical experience and data from a small series study [27] indicate these criteria are applicable in this age group with the caveats discussed in Macdonald et al. [24].
The D waves are insensitive to anesthetic agents and can be generated even in the presence of greater than 1 MAC of volatile agent [28]. While the amplitude of the D wave may not be affected, the exact latency of the D-wave may be sensitive to volatile agents. The I waves are highly sensitive to anesthetics [28] as well as to ischemia. Cortical ischemia produces an abrupt decrease in MEP amplitude of about 50–75 % due to loss of late I waves [29]. Cortical inhibitory circuits are immature in the infant and may lag excitatory circuits [30]; this may contribute to a reduction in I wave production with transcranial stimulation. The sensitivity of MEPs to anesthetic agents is due largely to the sensitivity of the αMNs. All anesthetics decrease the resting membrane potential of the αMNs; thus, a greater change in membrane potential is required to achieve depolarization [31]. The neuromodulatory influence of serotonin and noradrenaline has a significant effect on αMN excitability by regulating channel open-state time and resting membrane potential [32]. Volatile agents reduce serotoninergic output while ketamine may potentiate it [33, 34]. All of the adverse effects of anesthetic agents on MEP generation are more prominent in young children due to immaturity of the motor system. Although direct corticospinal tract to αMN connections are present in the MNs innervating muscles of the hand [35], there are both anatomic and molecular changes in the properties of the αMNs associated with maturation [36–38].
As a consequence of these factors, special techniques such as temporal or spatial facilitation may be required to elicit MEPs in very young children. The temporal facilitation technique, also called double-train stimulation, can be very effective for obtaining TcMEPs from young children [39, 40]. Spatial facilitation is technically more difficult and is limited to the homonymous muscle in a single (or two, upper or lower) limb(s), whereas temporal facilitation has no such limits. The stimulation parameters found to be effective in over 60 % of cases are shown in Table 43.2. The interstimulus interval is defined as the time between pulses in a train of pulses and has units of milliseconds. Some equipment manufacturers do not specify interstimulus interval per se, but rather specify rate in pulses per second. A rate of 1000 pulses per second yields an interstimulus interval of 1 ms. The intertrain interval is the time between trains. It is defined in the literature as the time between the start of the first train and the start of the second train. However, on some commercially available equipment, the definition is the time between the end of the first train and the start of the second train—the distinction is critical. The intertrain interval (ITI) and interstimulus interval (ISI) may require optimization depending on the target muscle group and any underlying pathology. The degree of facilitation is sensitive to the intertrain interval [39]; the maximum amplitudes of TcMEPs obtained from upper versus lower extremity muscles will generally occur at different interstimulus intervals, with longer ISIs favoring lower extremities [41].
Table 43.2
Parameters for use of temporal facilitation TcMEPs
Parameter | Value | Range | Comments |
---|---|---|---|
Voltage | Start 125/125 | As needed | Achieve MEP amplitude variance of < 20 % of mean |
Pulse length | 75 μs | 50–100 | 100 μs optimal but considered slow charge in US |
Pulses in train | 4/4 | 3/3–4/6 | Longer trains may elicit LE MEPs |
Interpulse intervala | 1–1.33 ms | 1–3 ms | Short IP(S)I favors UE and CN, longer favors LE |
Intertrain intervalb | 12 ms | 9–15 ms | Equipment-specific definition |
Electromyography
The basic principles of electromyography have been discussed in Chap. 7. The application of the technique to infants and children under anesthesia does not require the use of special techniques such as those required to elicit TcMEPs. The muscle mass of infants and young children is reduced compared to adults; at birth, the diameter of individual fibers is about one-fourth that of an adult. Fiber diameter increases in size through puberty [42]. Additionally, the mean duration of motor unit action potentials is significantly shorter in infancy and early childhood than at 20 years of age. Thus, EMG responses will have a different appearance and may have lower than expected amplitude, especially if subdermal needles are used. Positioning the needle electrodes intramuscularly will enhance the value of EMG monitoring [43].
Electroencephalography
The EEG waveform represents the fluctuating influence of excitatory postsynaptic potentials (EPSPs) and inhibitory postsynaptic potentials (IPSPs) on the dendrites of cortical neurons in part generated by the interaction of cortical and thalamo-cortical relay neurons. The frequencies observable from these recordings are limited by the skull, galea, and scalp as these structures form an effective low-pass filter. The EEG can be recorded and analyzed by direct inspection of waveform patterns and by means of the density spectral array (DSA). The EEG exhibits certain characteristics that are age and anesthetic agent dependent [44]. In general, there is a progression of the EEG frequency content from posterior to anterior—the fastest frequencies occurring in the frontal recording montages. The normal EEG displays symmetry between hemispheres and shows synchronization across montages. Sharp wave and spikes may suggest an underlying seizure disorder. Abnormally slow EEG suggests diffuse metabolic abnormalities. The anesthetic depth is determined by the proportion of alpha activity (8–15 Hz), beta activity (15–25 Hz), theta activity (4–7 Hz), and delta activity (1–3 Hz) recorded on the EEG tracing. Spectral edge frequency has been used as a marker of anesthetic depth although the correlation between sedation scores and SEF95 remain imperfect as is true for bispectral index (BIS) [45]. Children younger than 2 years of age, especially those younger than 12 months, display higher SEF95 values for similar sedation scores than older children; the same is true for BIS values [46].
For many procedures, a simple two-montage EEG such as Fp1-C3, Fp2-C4 may be sufficient. Procedures involving the cerebral vasculature may require use of more extensive EEG monitoring, using montages that cover the at-risk vascular territories and the watershed areas associated with the cerebral vasculature in question. As noted previously, much of the frontal EEG spectral power in a young child under anesthesia is in the delta frequency band. However, significant alpha frequency power may also present in children over 6 months of age. Loss of the alpha frequency power and generalized EEG amplitude reduction during vascular procedures or mass lesion resections near major vessels, unless associated with an abrupt change in anesthetic “depth,” or level, is cause for alerting the surgeon, analogous to similar changes during a carotid endarterectomy (see Chap. 20, “Monitoring Applications and Evaluating Changes”).
Brain Stem Auditory Evoked Responses
The neurophysiology of brainstem auditory evoked responses and the techniques for acquiring them are discussed in Chap. 3, “Auditory Evoked Potentials .” Auditory evoked responses are typically divided into short, middle, and long latency responses. During surgery, only the short latency responses are resistant to the effects of anesthetic agents and are useful for assessing the integrity of the eighth cranial nerve and the ascending auditory pathways to the level of the inferior colliculus. The “resistance” of short-latency BAERs to anesthesia is age dependent; clinical experience indicates that identification of wave V in infants younger than 6 months who have been anesthetized with sevoflurane is much more difficult, due to low amplitude signals, than in infants anesthetized with propofol (Unpublished data). In the nonanesthetized state, BAERs can be recorded from infants at 25 to 27 weeks’ gestation. Term infants will have wave I latencies similar to those of adults; wave III and V latencies reach adult values by 18–36 months of age [47].
Bulbocavernosus Reflex
Monitoring the bulbocavernosus reflex has been proposed by Skinner as a means of preserving lower sacral nerve function during intradural and extradural surgeries at the level of the conus medullaris, cauda equina, sacral plexus, and the pudendal nerve [48] (see Chap. 8, “The Use of Reflex Responses for Intraoperative Monitoring” for details). Acquisition of the reflex requires use of a multipulse technique applied as a double train with a long intertrain interval. Alternatively, a double-tap technique can be used. In either case, the acquisition time must be sufficiently long to capture the response (200 ms window). The reflex is also sensitive to anesthetic technique in young children and infants; TIVA is the preferred anesthetic technique for acquisition of this reflex. Recording electrodes are placed on opposite sides of the anal sphincter. These same electrodes can be used to record TcMEPs from the anal sphincter. Loss of the BCR but not the anal sphincter TcMEP suggests damage to the efferent pathways in the reflex arc. We record BCRs during complex tethered cord releases, lipoma resections, and similar procedures. The presence of a preexisting major urodynamic abnormality usually makes recording the BCR impossible, whereas mild abnormalities are not incompatible with recording the BCR (Fig. 43.2).
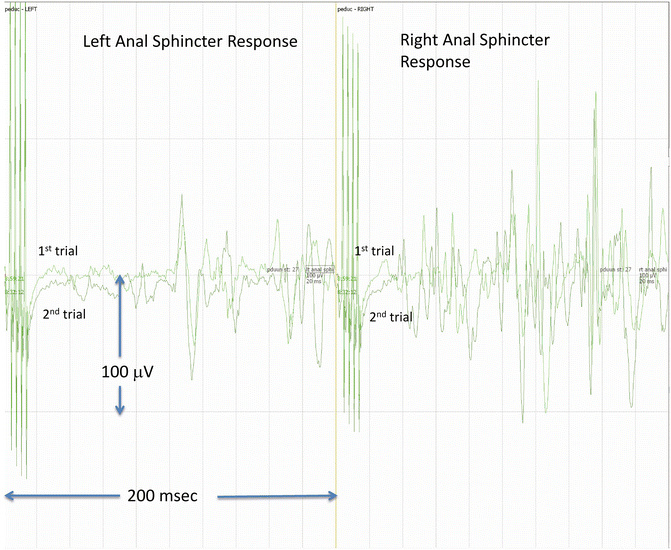
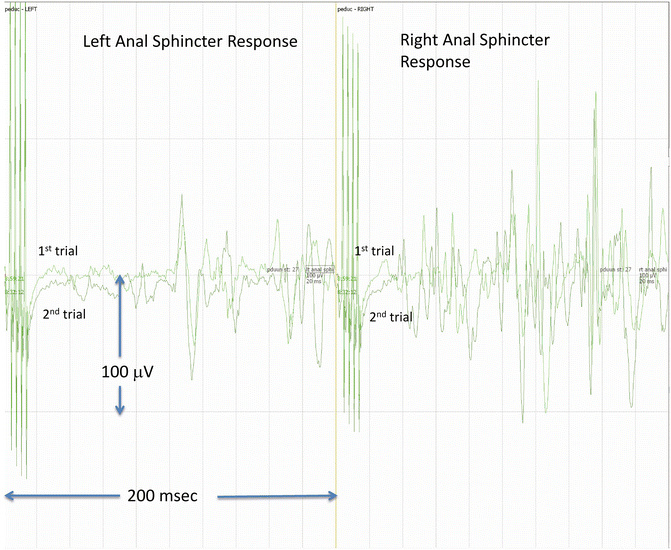
Fig. 43.2
Bulbocavernosus reflex (BCR) . The BCR was elicited from an 11-month-old girl undergoing repair of complex tethered cord and spinal cord lipoma resection. The stimulating cathode was placed at the clitoris and the anode slightly laterally and caudad. Correct positioning of the anal sphincter recording electrodes is also important (see Skinner reference for details). Two trials of right side stimulation are shown. In this case, a bilateral response is obtained. Note the complexity and duration of the response. The right side response appears to have two parts; loss of either the early or the late response may be significant and should be reported to the surgeon
Common Pediatric Surgical Procedures Utilizing IONM
A summary of common pediatric surgical procedures and the IONM modalities utilized during these procedures is presented in Table 43.3. A detailed discussion of some of the listed procedures follows.
Table 43.3
Common pediatric surgical procedures and IONM modalities
Procedure | Modalities | Comments |
---|---|---|
Posterior spinal fusion | UN & PTN SSEPs, TcMEPs EMG, trig-EMG | |
Spinal cord tumor resection | UN & PTN SSEPs, Dorsal column mapping, D wave, TcMEPs, EMG | D wave may not be obtained if child < 22 months. old or lesion below T10 |
Tethered cord and variants
![]() Stay updated, free articles. Join our Telegram channel![]() Full access? Get Clinical Tree![]() ![]() ![]() |