Intracranial Physiology and Elevated Intracranial Pressure
An understanding of the physiologic changes that result from the addition of a mass to the intracranial vault is essential to the treatment of mass lesions and cerebral edema, and to the effective use of intracranial pressure monitoring devices. Much of this understanding has been derived from the clinical work of Lundberg and colleagues with head injury patients in the 1960s (Chapter 12) and experimental observations of mass effect in animals. Since then, this early work has been supplemented by systematic study in patients with head injury and massive brain swelling after cerebral infarction. It is not always clear how some of this work pertains to day-to-day basis decisions in an intensive care unit. Perhaps the largest problem has been the focus on manipulating physiologic variables without a clear understanding of how they may alter outcome; this often leads to physiologically desirable results but no improvement in outcome. Nonetheless, the following exposition gives a basis for sensibly managing all patients with cerebral masses.
The intracranial vault serves to protect the brain, provide it with nutrients, and remove waste products. Several unique physiologic aspects of the intracranial compartment are central to understanding the disturbances that occur in various pathologic states. In this chapter we review: (a) the components of the intracranial compartment [brain, cerebrospinal fluid (CSF), intravascular blood]; (b) the manner in which these components are perturbed in disease states and the resultant clinical signs; (c) the concept of intracranial hypertension and the mechanisms whereby it produces secondary injury; and (d) the monitoring of intracranial pressure.
CONTENTS OF THE CRANIAL CAVITY
The skull is a rigid, almost completely closed container filled with brain, blood, and CSF. The brain, including its intracellular and interstitial fluid, fills 80% to 90% of the skull; the remainder is approximately equally divided between blood and CSF. The essential tenet of intracranial bulk physiology is that expansion of one compartment occurs at the expense of the others (the Monro-Kellie doctrine).
Brain
The brain floats in the CSF under normal conditions. Dural reflections, which form the falx cerebri and tentorium, act to maintain the position of the brain. In addition, they create compartments that, in pathologic states, produce pressure differentials within the cranium. The brain consists of approximately 78% water, 80% to 85% of which is in the intracellular compartment (1); the remainder is in the interstitial compartment. These relative distributions of water become important when considering osmotic therapies that differentially affect the intracellular and interstitial water compartments.
Pathologic states that increase the water content of the brain generally increase its volume. Cell death, which is followed by an increase in osmolality caused by the breakdown of cellular elements, leads to a shift of fluid from the interstitial to the intracellular compartment (“cytotoxic edema”) but causes no net change in brain volume. In contrast, disruption of the function of the blood-brain barrier (BBB) causes the overall water content of the brain to increase (“vasogenic edema”). These two mechanisms are operative to varying degrees in a wide variety of pathologic processes, including cerebral ischemia, trauma, and inflammation (1).
The brain, being virtually incompressible, is nonetheless capable of some degree of deformation as a consequence of pressure exerted by a mass within or external to it. Any further pressure causes the displacement of brain tissue, often into adjacent dural compartments, a circumstance called “herniation” by pathologists. These displacements correspond in some cases to particular clinical syndromes, as discussed in the following.
CEREBROSPINAL FLUID
Under normal conditions, the production of CSF is in dynamic equilibrium with its absorption. In the average adult there is 90 to 150 mL of CSF within the ventricular and subarachnoid spaces. The CSF volume in children (4 and 13 years of age) is 65 to 140 mL. Cerebrospinal fluid is produced at a rate of approximately 20 mL/h, originating predominantly in the choroid plexus (2). In addition, bulk flow of water from the interstitial fluid of the brain toward the lateral ventricles contributes to the CSF volume.
The production of CSF is depressed to a modest degree by elevated intracranial pressure (ICP), but it is usually unchanged in adults with most forms of hydrocephalus. Certain drugs, including acetazolamide (3), furosemide (4), and corticosteroids (5) are able to temporarily decrease CSF production in experimental and some clinical circumstances but they have no real clinical utility. Choroid plexus papilloma is the only important cause of overproduction of CSF; hydrocephalus usually results.
Absorption of CSF into the venous system is less well understood. It appears that the arachnoid villi are the main sites for reabsorption. These structures act as one-way valves between the subarachnoid space and the superior sagittal sinus that open at a gradient of about 5 mm Hg. In addition, some CSF leaks out around spinal nerve roots. Reabsorption increases to a slight degree as ICP rises.
The natural bulk flow of CSF originates in the lateral ventricles, descends through the third and fourth ventricles to exit by the foramina of Luschka and Magendie at the medullary level, and then percolates upward via the brainstem basal subarachnoid cisterns through the perimesencephalic cisterns at the tentorial plane and over the convexity of the cerebral hemispheres to reach the arachnoid villi. The flow is driven by the slight pressure gradient that exists between the ventricles and the convexity subarachnoid space and appears to be facilitated by the pulsatile activity transmitted from the vascular system.
The flow of CSF can be disturbed in a number of ways to produce hydrocephalus. All hydrocephalus is essentially “obstructive” at some site in the ventricular or subarachnoid pathways (with the exception of CSF overproduction from choroid plexus papilloma). However, the term obstructive hydrocephalus has come to denote a blockage to flow within the ventricular system as occurs, for example, when intraventricular blood or a shift of brain tissue because a cerebral mass blocks the ventricles at any one of a number of locations. Subarachnoid blood, meningitis, and neoplastic infiltration obstruct the egress of CSF from the fourth ventricle or, more commonly, at the basal cisterns or over the cerebral convexity or arachnoidal reabsorption sites. The latter type of hydrocephalus has been called “communicating” (a misnomer), but rectifying the frequent misuse of these terms seems futile.
CEREBRAL BLOOD FLOW AND VOLUME
The blood within the cerebral vessels is the third constituent of the intracranial space. The volume of intracranial blood under normal conditions is determined primarily by cerebral blood flow (CBF) and the cerebral vascular tone (6,7). Conditions that raise CBF generally raise cerebral blood volume (CBV) and conditions that lower CBF also lower CBV.
A number of physiologic factors influence CBF and thus CBV, some locally and others globally. The main clinically relevant factors are regional cerebral metabolism, global cerebral perfusion pressure (CPP), and the tensions of blood oxygen and carbon dioxide. The ability to retain normal cerebral blood flow through inherent adjustments in cerebral vascular diameter (tone) of small penetrating arterioles is termed autoregulation, as discussed in the following.
METABOLISM AND CEREBRAL BLOOD FLOW
Because the brain generates high-energy phosphates through the oxidative metabolism of glucose and there is no storage of this substrate, cerebral metabolism ultimately is dependent on the continuous delivery of glucose and oxygen by the blood (8). This circumstance is unique to the brain, yet its metabolic needs are considerably higher than other organs. Thus, although the brain accounts for only 2% to 3% of body weight, it receives 15% to 20% of the cardiac output. In the normal adult, CBF is approximately 50 to 75 mL/100 g per minute in the gray matter where metabolic needs are highest, and about half that level in white matter. Under normal conditions CBF is tightly regulated to match the global and regional metabolic needs of the brain, the primary determinant of regional CBF being the metabolic requirement of the cerebral cortex (9,10). The mechanism of this coupling is complex and involves the local effects of several metabolic products [K+, adenosine, adenosine triphosphate (ATP), pH] as well as an incompletely understood neural regulation of vascular tone. The coupling provides for enhanced CBF in response to, or in anticipation of, cortical metabolic needs and is reflected by reduced flow in damaged or inactive areas.
As mentioned, factors that affect cerebral metabolism generally produce parallel changes in CBF. For example, fever and seizure activity correspondingly increase CBF, CBV, and ICP. Indeed, the most striking elevations in CBF are seen during seizures, in which maximal neuronal activity is elicited. However, CBF and cerebral metabolism may become uncoupled in pathologic states. The nature of this uncoupling is not fully understood but may reflect factors that have such profound effects on blood flow and metabolism as to overwhelm the normal relationship. In these situations CBF appears to be influenced by other factors such as perfusion pressure, PaO2, arterial oxygen content (CaO2), PaCO2, and blood viscosity. All of these mechanisms are eclipsed in circumstances of greatly raised ICP, which ultimately reduces both CBF and CBV to levels that cause neuronal damage.
CEREBRAL PERFUSION PRESSURE AND AUTOREGULATION
Cerebral perfusion pressure is defined as the blood pressure gradient across the cerebral vasculature; it is an approximation that represents the difference between mean arterial pressure (MAP) at the entrance to the brain (Circle of Willis) and the venous pressure as its exits the skull. When ICP exceeds venous pressure, it replaces it in the calculation of CPP. For practical purposes, CPP is considered to be the mean arterial pressure (MAP) minus the mean ICP. When ICP and venous pressure are low, blood pressure is used to approximate CPP. Unless these measurements are made with the patient supine (in which case the brain and heart are at the same levels), all pressures can be referenced to head level, or appropriate account can be taken of the difference in altitude.
Cerebral blood flow remains constant over a fairly wide range of perfusion pressures in the absence of brain injury or other process that disrupts autoregulation (Fig. 2.1) (11, 12, 13 and 14). This allows the maintenance of constant brain perfusion over a similarly wide range of blood pressure and ICP changes. Autoregulation acts through changes in cerebrovascular resistance (CVR), specifically by altering the diameter of arterioles (12). As perfusion pressure falls, the arterioles apparently dilate to maintain CBF, thereby increasing CBV. Cerebral vasoconstriction occurs with increasing perfusion pressure, and CBF is maintained with a lower CBV. The upper and lower limits of autoregulation in healthy normotensive adults are approximately 50 and 150 mm Hg. Both the upper and lower limits of autoregulation are elevated in chronically hypertensive patients, but they tend to normalize with long-term blood pressure control (15). Although the limits of autoregulation are not known for children, autoregulation may be intact at lower absolute values of blood pressure in small children. Abrupt, pronounced elevations in blood pressures that exceed the upper limits of autoregulation result in increased CBF and when extreme, edema and hemorrhage. Outside the limits of autoregulation, CBF becomes passively dependent on changes in perfusion pressure. In this case, CBF and CBV fluctuate passively in the same direction.
Autoregulation is maintained through a combination of local myogenic control of arteriolar resistance and sympathetic neural activity. Sympathetic tone appears primarily to affect the upper and lower limits of autoregulation. Increased sympathetic activity during hypotension increases cerebrovascular tone and raises the threshold for blood pressure breakthrough (16). It should be noted that autoregulation may lag behind changes in blood pressure by 1 to 2 minutes, particularly in the damaged region of brain, allowing acute hypertension to raise ICP momentarily. Prolonged hypotension has been shown to impair autoregulation even after blood pressure has been restored (10,17).
Autoregulation is impaired or lost under a number of pathological states, including ischemic stroke and head injury (18, 19, 20 and 21). Perhaps
of even greater importance, autoregulation is more effective in maintaining normal CBF when ICP is elevated than when blood pressure is reduced (22,23); restated, low perfusion pressure that results from systemic hypotension theoretically is a greater risk than the equivalent CPP that results from high ICP.
of even greater importance, autoregulation is more effective in maintaining normal CBF when ICP is elevated than when blood pressure is reduced (22,23); restated, low perfusion pressure that results from systemic hypotension theoretically is a greater risk than the equivalent CPP that results from high ICP.
![]() FIG. 2.2. Relationship between arterial oxygen content and cerebral blood flow (top) and arterial partial pressure of oxygen and cerebral blood flow (bottom). See text for explanation. |
Oxygen Tension
The relationship between CBF and oxygen is best understood when considered in terms of arterial oxygen content (CaO2) (Fig. 2.2). CaO2 is measured as
1.34 × ([hemoglobin] × O2 saturation) + (PaO2 × 0.003)
The two components of this equation account for the oxygen that is bound to hemoglobin and O2 dissolved in plasma, respectively. Because the amount of oxygen dissolved in plasma is small, practically speaking, CaO2 is proportional to hemoglobin concentration times O2 saturation. The relationship between CaO2 and CBF is linear; thus, CBF rises dramatically with anemia (24). Because of the shape of the hemoglobin dissociation curve, CaO2 changes little until PaO2 falls below about 50 mm Hg; thus, CBF is constant within a wide range of arterial oxygen pressure (Fig. 2.1) (25,26).
Carbon Dioxide Tension
Cerebral blood flow is sensitive to arterial carbon dioxide pressure (PaCO2) in the physiologic range of the latter (Fig. 2.3). There is a linear relationship between PaCO2 and CBF for PaCO2 between approximately 20 and 80 mm Hg (27, 28, 29 and 30). Lowering PCO2 from 40 to 20 mm Hg reduces CBF by 40%, whereas raising PCO2 to 80 mm Hg nearly doubles CBF (31). When intracranial compliance is high, the added volume is buffered by CSF translocation. In states of poor compliance, marked elevations in ICP can result from even modest hypercarbia (32). It appears that carbon dioxide vascular reactivity is mediated by arteriolar and brain extracellular fluid hydrogen ion concentration (33). Cerebral blood flow is normally reduced for several hours after a single-step elevation in CSF pH, the same time required for correction of CSF alkalosis (34). Persistent hypercarbia in normal brain causes an elevation in CBF and ICP lasting less than 12 hours (35, 36 and 37). Theoretically, profound hypocapnia could produce sufficient vasoconstriction to reduce blood flow below the level necessary to supply metabolic needs and cause ischemia. However, recent studies have challenged this assumption and suggest that short-term hyperventilation is probably safe (38), although it has not been shown to improve overall outcome. This ability of hypocarbia to reduce CBF and CBV and thereby to reduce ICP is the basis for therapeutic use of hyperventilation (Chapter 3).
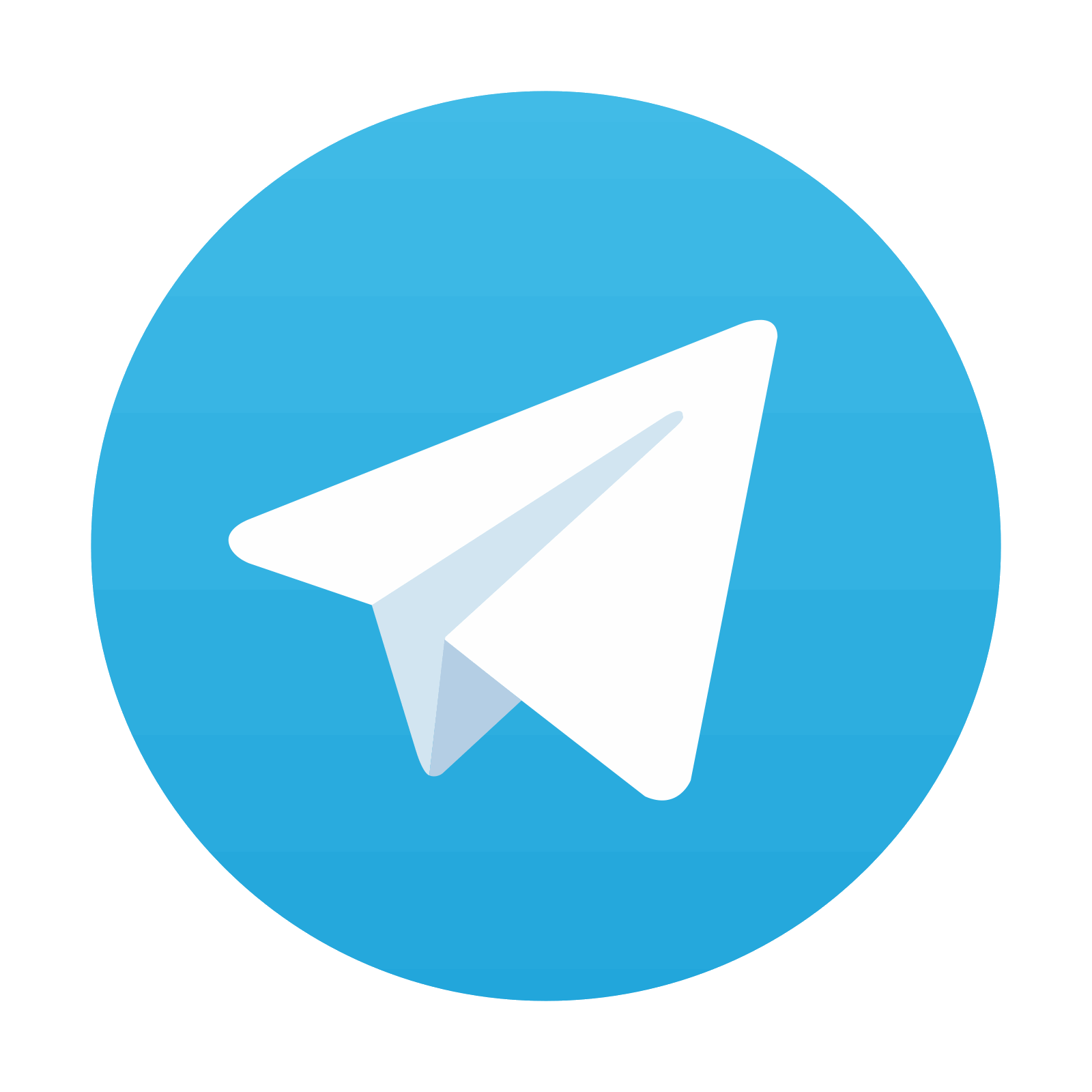
Stay updated, free articles. Join our Telegram channel
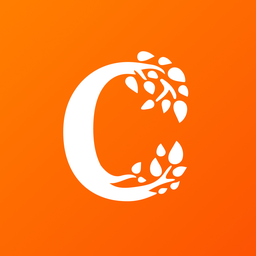
Full access? Get Clinical Tree
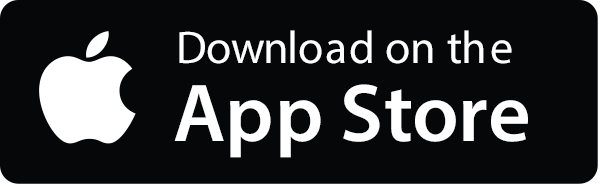
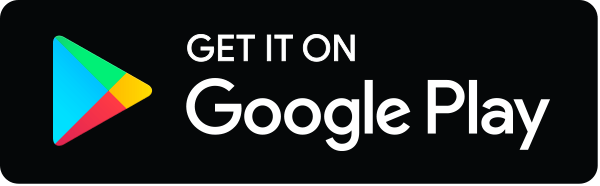