Inhaled Gases and Noninvasive Ventilation
Angela T. Wratney
Donna S. Hamel
Ira M. Cheifetz
David G. Nichols
KEY POINTS
Oxygen


Nitric Oxide




Helium-Oxygen Mixtures
Bronchodilator Therapy



Inhalational Anesthetics


Noninvasive Ventilation



The provision of inhaled gases and the use of noninvasive respiratory support provide therapeutic resources fundamental to the management of critical care illness. The clinical and pathophysiologic processes associated with respiratory failure are directly targeted by medical gases used in the PICU (see also Chapters 46, 47, 48, 49, 50). Therefore, critical care personnel must be familiar with the following inhaled medical gases and medications: (a) oxygen (O2), (b) inhaled nitric oxide (iNO), (c) helium-oxygen mixtures (heliox), (d) inhaled bronchodilators, and (e) inhalational anesthetics. In addition, noninvasive ventilation (NIV) offers a valuable alternative to intubation and mechanical ventilation for many critically ill patients. This chapter includes a discussion of the pharmacologic and therapeutic mechanisms of inhaled gases, the appropriate delivery system technology, and important safety considerations for each of these therapies used in the PICU.
HUMIDIFICATION
Humidification is an extremely important component of inhaled gas administration in the pediatric population. The nasal passages and upper airway warm, humidify, and filter air for the respiratory system. Thus, in the provision of inhaled gas therapies that bypass the upper airway or are administered with high pressure or flow, careful attention to temperature
control, humidification, and infection control is warranted. Failure to do so may result in hypothermia, inspissated secretions, and airway injury and potentially contribute to the development of ventilator-associated pneumonia (1).
control, humidification, and infection control is warranted. Failure to do so may result in hypothermia, inspissated secretions, and airway injury and potentially contribute to the development of ventilator-associated pneumonia (1).
OXYGEN
The fraction of inspired oxygen (FIO2) in ambient air is 0.21, which is commonly expressed as a percentage (21%). For medical use, 100% oxygen is mass produced from fractional distillation of atmospheric air. O2 is provided to patients with impaired respiratory function to improve arterial O2 saturation, to vasodilate the pulmonary capillary bed, and to enhance systemic oxygen delivery.
Energy Metabolism
O2 is essential for cellular aerobic metabolism. Oxidative phosphorylation of NADH within the mitochondria generates 36 moles of ATP for every mole of glucose consumed. Under anaerobic conditions, glycolysis rapidly produces lactic acid as well as only 2 moles of ATP per mole of glucose. The resultant intracellular acidosis ultimately inhibits the enzymes involved in glycolysis.
Physiologic Mechanisms
Supplemental O2 is administered to the hypoxemic patient to increase alveolar O2 tension, which in turn raises arterial O2 tension and saturation, thereby enhancing systemic O2 delivery to the tissues. Arterial O2 tension may fail to rise despite supplemental O2 for the following reasons:
Hypoventilation (e.g., oversedation).
Decreased ventilation-perfusion ([V with dot above]/[Q with dot above]) matching in the lung (e.g., pneumonia, atelectasis). Low mixed venous O2 saturation magnifies hypoxemia from decreased [V with dot above]/[Q with dot above] ratios. The mixed venous O2 saturation is reduced when systemic O2 demand exceeds O2 supply (e.g., shock, anemia).
Anatomic right-to-left shunt (e.g., cyanotic congenital heart disease).
See Chapter 43 on Respiratory Physiology for more detail on these concepts.
Oxygen Delivery Systems
Depending on the desired FIO2, a variety of devices exist for administering supplemental O2 (Table 39.1). The flow pro
vided by the O2 delivery system will be either sufficient to meet the inspiratory flow demand of the patient (high-flow systems) or insufficient (low-flow systems), causing the patient to entrain additional room air flow. High-flow delivery systems provide a more stable, measurable delivery of the set FIO2, independent of patient respiratory effort. High-flow delivery devices can be used with face masks with a reservoir (non-rebreather or partial rebreather), tracheostomy collars, nebulizers, O2 hoods, and specialized high-flow nasal cannula (HFNC) systems. Low-flow systems include nasal cannulae or simple face masks (without reservoirs).

TABLE 39.1 FRACTION OF INSPIRED OXYGEN PROVIDED BY VARIOUS DELIVERY SYSTEMS | ||||||||||||||||||
---|---|---|---|---|---|---|---|---|---|---|---|---|---|---|---|---|---|---|
|
Nasal Cannulae
The nasal cannulae provide a comfortable, lightweight, and inexpensive method for low-flow O2 delivery (0.1-6 L/min). FIO2 delivery ranges between 24% and 50%. Although highly variable in practice, a general “rule of thumb” states that for each liter of supplemental O2 flow, the inspired O2 concentration increases by ˜4% (2, 3). Rapid respiratory rates, higher tidal volumes, and shorter inspiratory times cause increased air dilution and reduce the actual inspired FIO2. Two caveats bear mentioning for use of nasal cannulae in the infant: (a) when administering low flow (e.g., flow <1 L), the actual flow and FIO2 provided may be highly inaccurate because of the frequent occurrence of system leaks, displaced nasal prongs, and the limitations in flow meter calibration; and (b) when administering higher flow (e.g., >2 L), the infant may receive significant positive end-expiratory pressure (PEEP) with the attendant risks of barotrauma (3, 4). Heating and humidification of the nasal cannula O2 flow are mandatory with higher flow rates (>2 L/min) to prevent nasal drying, irritation, and heat loss.
Face Masks
Simple face masks fit over the patient’s nose and mouth, providing an FIO2 delivery of between 35% and 55% O2. A nonrebreathing face mask consists of a simple face mask adapted with a reservoir bag and an inflow system for fresh gas infusion to increase FIO2 delivery to close to 100% O2. Two one-way valves, located between the mask and the reservoir and at one of the side exhalation ports, ensure that each inspiratory breath consists of fresh gas without entrainment of room air and that exhaled gas is eliminated into the environment rather than the reservoir bag. As a safety mechanism, the other side exhalation port is kept open to allow the entrainment of room air in the event that gas flow to the mask system is interrupted. Similarly, a partial rebreathing mask consists of a mask with an attached reservoir bag and an inflow gas source. However, the system lacks the unidirectional valve between the face mask and the reservoir. Thus, it allows the mixing of the exhaled CO2 gas from the patient with the O2 in the reservoir bag. To avoid rebreathing exhaled CO2, the inspired gas flow rate should be maintained at or above 6 L/min.
Venturi masks are high-flow systems that provide fixed concentrations of 24%, 28%, 31%, 35%, 40%, or
50% O2. These concentrations are obtained by delivering manufacturer-recommended flow rates necessary to generate specific oxygen-to-air entrainment ratios. The flow velocity of O2 entering the mask causes the entrainment of air through side ports in the device. Dependable O2 concentrations are administered as long as total gas flow exceeds the patient’s peak inspiratory flow rate.
50% O2. These concentrations are obtained by delivering manufacturer-recommended flow rates necessary to generate specific oxygen-to-air entrainment ratios. The flow velocity of O2 entering the mask causes the entrainment of air through side ports in the device. Dependable O2 concentrations are administered as long as total gas flow exceeds the patient’s peak inspiratory flow rate.
TABLE 39.2 REACTIVE OXYGEN SPECIES AND THE ENDOGENOUS ANTIOXIDANT DEFENSE SYSTEMS | ||||||||||||
---|---|---|---|---|---|---|---|---|---|---|---|---|
|
Oxygen Hood and Tent
O2 hoods and tents are transparent enclosures designed to surround the head or entire body of a small infant. Neither is commonly used today because they create access barriers to the infant, make observation of the infant more difficult, and may result in nonuniform (layered) O2 distribution inside the enclosure (5). Hoods can provide 80%-90% FIO2 with high flow of humidified O2 (10-15 L/min), whereas tents typically achieve up to 50% FIO2 with high O2 flow.
Blow-by
“Blow-by” O2 delivery involves wafting humidified O2 at high flow rates through the corrugated tubing toward the face. This is especially useful for anxious toddlers who require <30% FIO2 of fully humidified oxygen, because the caregiver can easily hold the child while directing the O2 flow toward the child’s face.
Risks
Oxygen Toxicity


The mechanism underlying oxygen toxicity involves the creation of reactive oxygen species (ROS), such as the superoxide anion (O2–), O2 radical (O2•), hydroxyl radical (OH•), and hydrogen peroxide (H2O2). ROS cause oxidative injury to proteins, DNA, and lipids. Organs rich in lipids and proteins, such as the pulmonary and central nervous system, are especially at risk. Endogenous antioxidant enzyme systems, such as superoxide dismutase (SOD), glutathione peroxidase, and catalase, attempt to clear ROS to limit cellular injury (Table 39.2). SOD converts superoxide and hydrogen to O2 and H2O2, whereas catalase detoxifies this product into H2O and O2. Glutathione peroxidase binds oxygen radicals with lipids to produce lipid hydroxides and water. Additional endogenous ROS scavengers include cholesterol, α-tocopherol (vitamin E), ascorbic acid (vitamin C), selenium, and bilirubin.
Antioxidant defense systems may be overwhelmed when exposed to high FIO2 or during prolonged O2 exposure. Individual patient susceptibility to O2 toxicity may derive from variations in the duration and fractional percent of O2 exposure, the underlying disease state, antioxidant availability, and individual genetic variations (7, 8).
Hypercapnia
Administering oxygen to patients with a chronically compensated respiratory acidosis may induce a mild increase in measured blood PaCO2. A similar increase in PaCO2 in response to exogenous oxygen administration has been seen in patients with neuromuscular disease, chronic asthma, and obesity hypoventilation syndrome. Hypercarbia is hypothesized to occur secondary to (a) loss of hypoxic pulmonary vasoconstriction, (b) decreased stimulation of peripheral chemoreceptors resulting in reduced ventilatory drive, and (c) the decreased CO2-binding affinity of oxyhemoglobin (i.e., the Haldane effect) (9, 10). This effect is minimal in clinically hypoxemic patients and should never preclude the administration of oxygen. However, vigilance is always necessary because the underlying diseases may progress, necessitating assisted ventilation.
INHALED NITRIC OXIDE
Named “Molecule of the Year” by Science magazine in 1992, endogenous nitric oxide (NO) is an essential gaseous cell mediator in neurotransmission, inflammatory cell activation, and vascular smooth muscle relaxation. Therapeutically, inhaled NO (iNO) is used as a selective pulmonary vasodilator to reduce pulmonary vascular resistance and treat pulmonary arteriolar hypertension (see Chapter 80). Although iNO has also been used to treat hypoxemic respiratory failure by improving [V with dot above]/[Q with dot above] matching, this therapeutic approach does not alter clinical outcomes and is very expensive.
Pharmacology
Endogenous NO is synthesized within most cells of the human body from arginine and O2 by one of three isoforms of NO synthase (NOS): neural, inducible, and endothelial. Constitutive NO production is found in the vascular endothelium, neurons, platelets, adrenal medulla, and macula densa of the
kidney. NO is also produced under pathologic conditions by the inducible NOS found within macrophages, hepatocytes, and airway epithelial and smooth muscle cells.
kidney. NO is also produced under pathologic conditions by the inducible NOS found within macrophages, hepatocytes, and airway epithelial and smooth muscle cells.
NO induces upregulation of cytosolic guanylyl cyclase, triggering the activation of cyclic guanosine 3′,5′-monophosphate (cGMP)-dependent protein kinases (Fig. 39.1). Ultimately, this cascade results in smooth muscle cell relaxation and pulmonary arteriolar vasodilation due to decreased intracellular calcium levels. Phosphodiesterase enzymes cause cGMP levels within the smooth muscle cell to fall. Phosphodiesterase enzyme inhibitors (e.g., sildenafil, zaprinast, and dipyridamole) preserve the cGMP-dependent cascade and may provide a synergistic increase in the vasodilatory effects of iNO.


![]() FIGURE 39.1. iNO causes pulmonary vascular endothelial smooth muscle cell relaxation, induces pulmonary capillary smooth muscle cell relaxation, and induces upregulation of guanylyl cyclase, resulting in cytosolic increase of cyclic guanosine 3′,5′-monophosphate (cGMP). cGMP- dependent protein kinase (cGKI) ultimately lowers the intracellular calcium concentration and decreases the sensitivity of myosin to calcium-induced contraction. (Adapted from Griffiths MJD, TW Evans. Drug therapy: Inhaled nitric oxide therapy in adults. N Engl J Med 2005;353:2683-95.) |
NO can also react with free radicals when available (Fig. 39.2) to form nitrogen dioxide (NO2) or peroxynitrite (ONOO–). Protein nitration and oxidative lung injury can result from these toxic reactions and may underlie the pathologic mechanism for hyperoxic or ischemia- reperfusion injury (13). The biochemical fate of NO may depend upon the relative concentrations of the various blood components (oxyhemoglobin, iron, and plasma proteins), ROS, and antioxidants.
![]() FIGURE 39.2. The biochemical fates of inhaled nitric oxide. Systemic uptake of iNO is limited by nitric oxide binding to oxyhemoglobin, heme iron, and plasma proteins. Depending upon the relative concentration of O2 and free radicals (reactive oxygen species), nitrogen dioxide (NO2) and reactive nitrogen species (i.e., peroxynitrite) may be produced. (Adapted from Griffiths MJD, TW Evans. Drug therapy: Inhaled nitric oxide therapy in adults. N Engl J Med 2005;353: 2683-95.) |
Nitric Oxide Delivery Systems
NO gas is produced at high temperatures by a catalyst-induced oxidation of ammonia and is then stored with nitrogen as the balancing gas. iNO is generally delivered during mechanical ventilation, but can also be delivered to nonintubated patients via a tight-fitting face mask, transtracheal O2 catheter, or nasal cannula. Effective iNO delivery via nasal cannula has been validated for flow rates as low as 1 L/min. iNO should not be administered via an O2 hood owing to the potential for accumulation of toxic nitrogen dioxide byproducts within the hood.
During mechanical ventilation, a constant desired concentration of iNO is delivered to the patient, independent of ventilator mode or flow rate. An integrated iNO delivery unit and nitrogen dioxide-monitoring system, such as the Ohmeda INOvent Nitric Oxide delivery system (Datex-Ohmeda, Madison, WI), is generally used. With this system, the minimum delivered concentration of iNO is 0.1 ppm, at a flow rate between 4 and 120 L/min. iNO is injected directly into the inspiratory limb of the ventilator circuit in synchrony with each inspiratory breath. iNO has an effective half-life of 15-30 seconds. Therefore, to ensure that the iNO flow is not interrupted in the event the patient must be disconnected from the ventilator, a separate connector is used to facilitate iNO delivery via a manual bag system. iNO delivery with the Bunnell Life Pulse High-Frequency Jet ventilator has been used in the clinical setting for premature infants with respiratory distress syndrome and has been tested with infant lung models (14).
NO Metabolite Monitoring
During iNO administration, the appropriate monitoring and measurement of NO2 and methemoglobin levels are necessary to prevent potential NO-induced toxicity. NO2 results from the reaction of NO with O2, and its level is continuously measured using electrochemical sensors. When administering iNO at doses <40 ppm, toxic levels of NO2 (>2 ppm) rarely occur (15, 16).
Methemoglobin is produced from NO reaction with oxyhemoglobin, which converts the iron to the ferric or oxidized state (Fe3+). Normally, methemoglobin is converted within the red cell by NADH-cytochrome b5 reductase to restore ferrous hemoglobin (Fe2+). Methemoglobinemia may result in patients with inefficient methemoglobin reductase activity or in those who are exposed to high concentrations of iNO. Blood methemoglobin levels should be monitored within 4 hours of initiating iNO and after every 24 hours of continued treatment. Prilocaine local or topical anesthetics are contraindicated in patients receiving iNO because of the risk of methemoglobinemia.
NO Dosing
Dosing and duration of iNO therapy may depend on the therapeutic target defined for each patient (i.e., improved [V with dot above]/[Q with dot above] matching versus decreased pulmonary arterial pressure). The effective dose of iNO may depend on the degree of reversible pulmonary arterial hypertension, the extent of [V with dot above]/[Q with dot above] mismatching, and the concurrent level of alveolar recruitment. iNO doses between 5 and 20 ppm should produce a clinically apparent response in oxygenation and/or pulmonary vascular resistance. Continuous iNO of >40 ppm does not produce greater benefit; rather, it can produce increased measured levels
of methemoglobin and nitrogen dioxide. In patients with hypoxemic respiratory failure, alveolar derecruitment has been associated with a poor response to iNO therapy at recommended doses. In patients with pulmonary hypertension, a decrease in pulmonary vascular resistance by 30% during a trial of 10 ppm iNO for 10 minutes has been predictive of those likely to derive a clinical response to oral agents (17). A systematic review of the literature fails to support the benefit of iNO on important clinical outcomes in ARDS, such as number of ventilator-free days or survival (18).

NO Weaning

HELIUM-OXYGEN MIXTURES (HELIOX)
Helium is administered clinically as a helium-oxygen mixture, referred to as heliox. Although helium is biologically inert and provides no direct pharmacologic or biologic effects, heliox
provides a medical gas therapy with unique therapeutic application to respiratory processes associated with high airways resistance or obstructive pathology (25). Owing to its low density, heliox can significantly decrease respiratory distress and work of breathing, and may improve the deposition of bronchodilator therapy to obstructed lower airways. Heliox provides a rapidly acting inhaled therapy that may afford the patient greater comfort and improved gas exchange while awaiting the therapeutic onset of slower, definitive medical therapies (e.g., corticosteroids).
provides a medical gas therapy with unique therapeutic application to respiratory processes associated with high airways resistance or obstructive pathology (25). Owing to its low density, heliox can significantly decrease respiratory distress and work of breathing, and may improve the deposition of bronchodilator therapy to obstructed lower airways. Heliox provides a rapidly acting inhaled therapy that may afford the patient greater comfort and improved gas exchange while awaiting the therapeutic onset of slower, definitive medical therapies (e.g., corticosteroids).
Because of small sample sizes, variable helium concentrations, and poorly controlled effects of helium on other inhaled bronchodilators, the quality of the evidence for using heliox in
specific diseases is generally poor. A recent systematic review of heliox therapy in viral croup concluded that heliox did not confer a significant benefit compared to humidified O2 or racemic epinephrine, but did appear to benefit children short-term (90-24 min) while awaiting the onset of the effects of dexamethasone (26). One small study indicated an advantage of heliox over racemic epinephrine in the treatment of postextubation stridor (27). For acute asthma exacerbations, small studies have suggested enhanced bronchodilator deposition with heliox inhalation (28
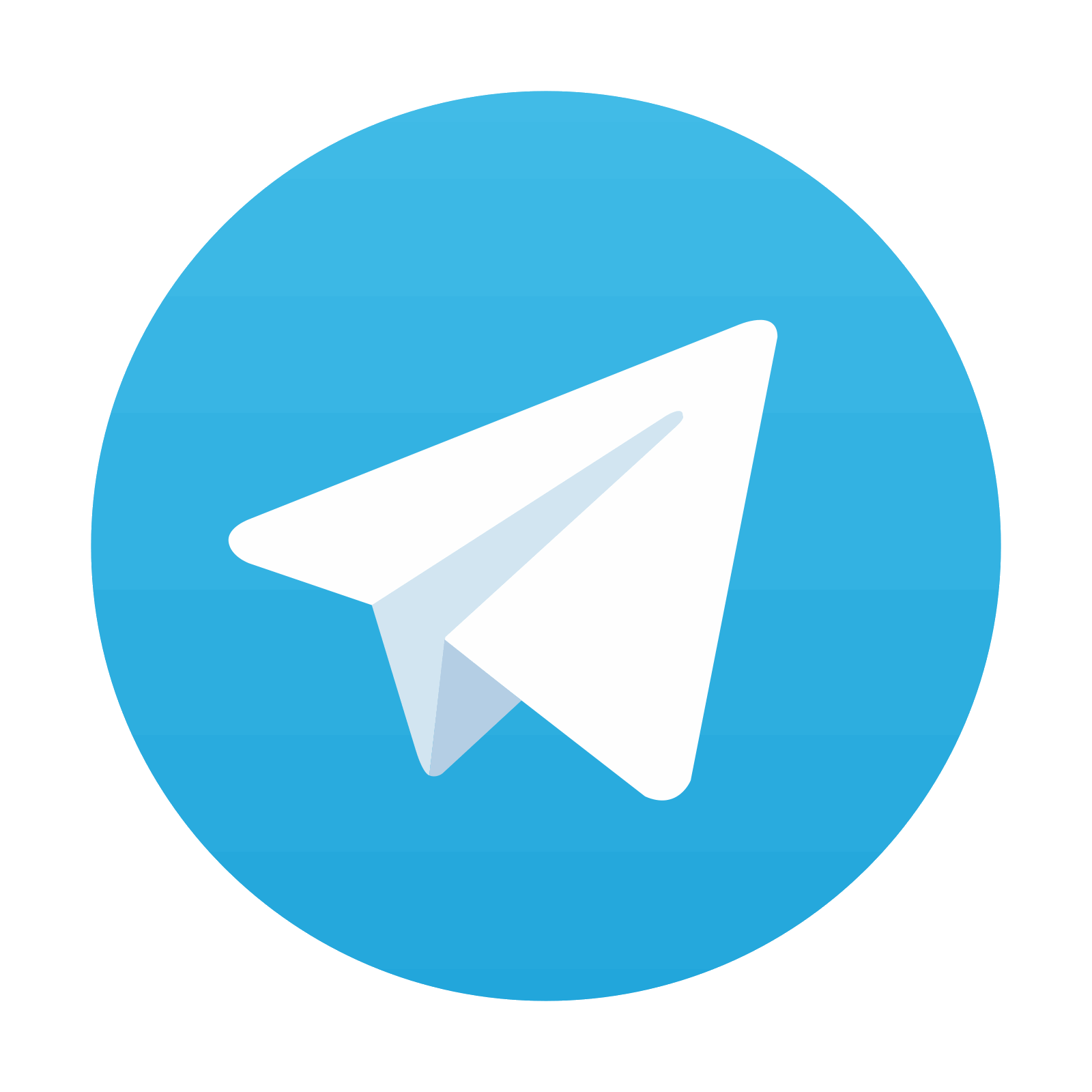

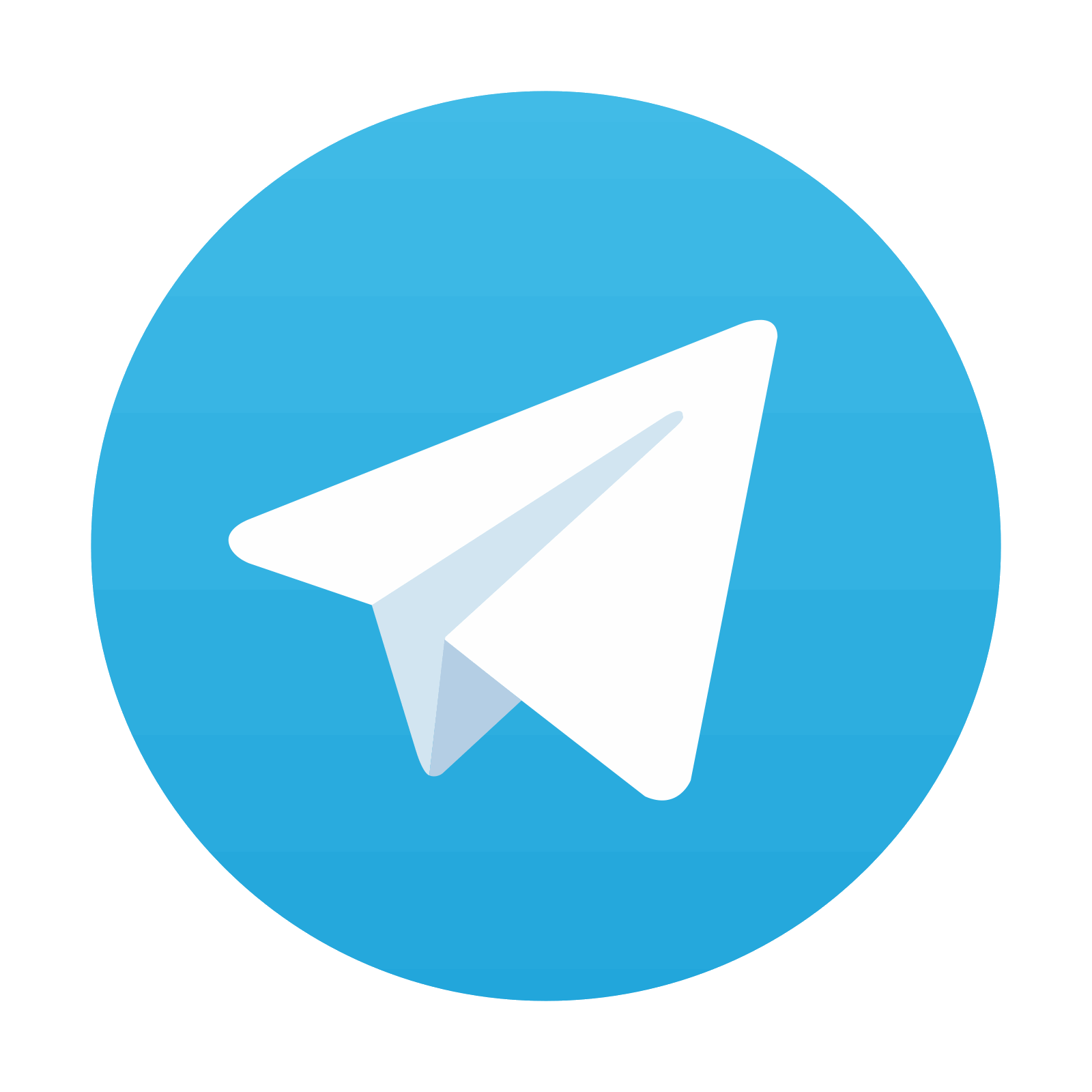
Stay updated, free articles. Join our Telegram channel
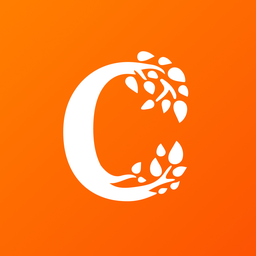
Full access? Get Clinical Tree
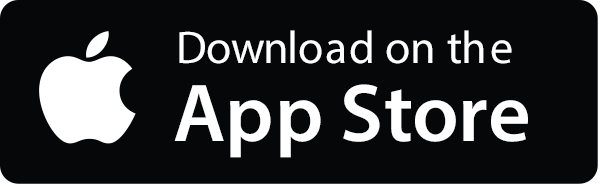
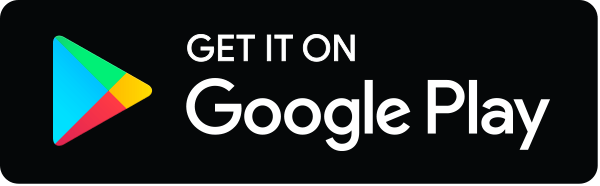