History
The discovery of inhaled anesthesia reflects the contributions of clinicians and scientists in the United States and England ( Fig. 7.1 ). The most commonly used inhaled anesthetics in modern anesthesia include volatile liquids (i.e., halothane, enflurane, isoflurane, desflurane, and sevoflurane) and a single gas (i.e., nitrous oxide) ( Figs. 7.2 and 7.3 ). Halothane, enflurane, and isoflurane are no longer commonly used. Yet, none of these inhaled anesthetics meets all the criteria of an “ideal” inhaled anesthetic, and the chemical characteristics differ among the drugs ( Table 7.1 ).



Characteristic | Isoflurane | Enflurane | Halothane | Desflurane | Sevoflurane | Nitrous Oxide |
---|---|---|---|---|---|---|
Partition coefficient | ||||||
Blood-gas | 1.46 | 1.9 | 2.54 | 0.45 | 0.65 | 0.46 |
Brain-blood | 1.6 | 1.5 | 1.9 | 1.3 | 1.7 | 1.1 |
Muscle-blood | 2.9 | 1.7 | 3.4 | 2.0 | 3.1 | 1.2 |
Fat-blood | 45 | 36 | 51 | 27 | 48 | 2.3 |
MAC (age 30-55 years) % of 1 atmosphere | 1.15 | 1.63 | 0.76 | 6.0 | 1.85 | 104 |
Vapor pressure at 20° C (mm Hg) | 240 | 172 | 244 | 669 | 160 | |
Molecular weight (g) | 184.5 | 184.5 | 197.4 | 168 | 200 | 44 |
Stable in hydrated CO 2 absorbent | Yes | Yes | No a | Yes | No a | Yes |
Stable in dehydrated CO 2 absorbent | No | No a b | No b | No a b c | Yes | |
Percent metabolized | 0-0.2 | 15-40 | 0-0.2 | 5-8 |
The First Inhaled Anesthetics
Nitrous Oxide
Nitrous oxide gas was first synthesized in 1772 by the English chemist, author, and Unitarian minister Joseph Priestley. Twenty-seven years later, Sir Humphry Davy administered nitrous oxide for dental analgesia. Although he suspected that nitrous oxide might be used to relieve pain for surgery, it was not until it was used 42 years later by a 29-year-old dentist named Horace Wells who administered nitrous oxide to himself and found that it relieved his pain. Specifically, he noticed the hypnotic and analgesic effects of nitrous oxide at a public exhibition in Hartford, Connecticut, in 1842. The next day, Wells himself underwent a dental extraction by a fellow dentist. Wells felt only minimal pain with the extraction, and he subsequently learned the method of nitrous oxide synthesis to make it available to his own patients. Two years later, he arranged to demonstrate painless dental surgery using nitrous oxide administration at the Massachusetts General Hospital. Not being completely successful, Wells was discredited as a result of this demonstration.
Diethyl Ether
William Morton, a Boston dentist, noticed that diethyl ether, during “ether frolics” in which ether was breathed for its inebriating effects, had similar effects as nitrous oxide. Like Wells, Morton applied ether in his dental practice and then demonstrated its anesthetic properties at the Massachusetts General Hospital on October 16, 1846 (“ether day”). In contrast to Wells’s debacle, Morton’s demonstration was received with great enthusiasm. The results of successful ether anesthetics were soon published in the Boston Medical and Surgical Journal . Although Crawford Long administered diethyl ether to a patient in 1842, 4 years earlier than Morton, he did not publicize his work, and Morton therefore has traditionally been credited with the discovery of diethyl ether’s capability to produce anesthesia.
Chloroform
James Simpson, an obstetrician from Edinburgh, Scotland, developed chloroform, which did not share the protracted induction, flammability, and postoperative nausea seen with diethyl ether. Chloroform soon became popular as an inhaled anesthetic in England, although diethyl ether dominated medical practice in North America. Unfortunately, chloroform was associated with several unexplained intraoperative deaths of otherwise healthy patients and numerous cases of hepatotoxicity.
Inhaled Anesthetics Between 1920 and 1940
Between 1920 and 1940, ethylene, cyclopropane, and divinyl ether were introduced into use as anesthetics, gaining acceptance over the older inhaled anesthetics (with the exception of nitrous oxide) by producing a faster, more pleasant induction of anesthesia and by allowing faster awakening at the conclusion of surgery. However, each had serious drawbacks. Many were flammable (i.e., diethyl ether, divinyl ether, ethylene, and cyclopropane), whereas others, halogenated entirely with chlorine, were toxic (i.e., chloroform, ethyl chloride, and trichloroethylene).
Fluorine Chemistry and Modern Inhaled Anesthetics
Techniques of fluorine chemistry, developed from efforts to produce the first atomic weapons, found a fortuitous, socially beneficial purpose in providing a method of synthesizing modern inhaled anesthetics. Modern inhaled anesthetics are halogenated partly or entirely with fluorine (see Fig. 7.2 ). Fluorination provides greater stability and lesser toxicity.
Halothane
Halothane was introduced into clinical practice in 1956 and became widely used. It had several advantages compared with the older anesthetics, including nonflammability, a pleasant odor, lesser organ toxicity, and pharmacokinetic properties allowing a much faster induction of anesthesia and emergence compared with ether. Unfortunately after 4 years of commercial use, reports of fulminant hepatic necrosis after halothane anesthesia began to appear in patients in which other causes of liver damage were not evident. The issue of unpredictable liver damage stimulated the search for other volatile anesthetics. Halothane also sensitizes the myocardium to the dysrhythmogenic effects of catecholamines.
Methoxyflurane
Methoxyflurane was first introduced into clinical practice in 1960. Within the first decade of its introduction, reports of renal failure with methoxyflurane anesthesia appeared, leading to studies confirming a dose-related nephrotoxicity because of the inorganic fluoride that resulted from the metabolism of this anesthetic.
Enflurane
Enflurane was introduced to clinical practice in 1972. Unlike halothane, it did not sensitize the heart to catecholamines and was not associated with hepatotoxicity. However, enflurane was metabolized to inorganic fluoride and could cause evidence of seizure activity on the electroencephalogram (EEG), especially when administered at high concentrations and in the presence of hypocapnia.
Isoflurane
Isoflurane was introduced into clinical practice in 1980 and was widely used clinically. It was not associated with cardiac dysrhythmias. Because it is not metabolized as readily as halothane and enflurane, isoflurane was associated with less toxicity. Isoflurane allowed a more rapid onset of surgical anesthesia and faster awakening compared with its predecessors.
Sevoflurane and Desflurane
Sevoflurane and desflurane are halogenated exclusively with fluorine and were first synthesized during the late 1960s and 1970s, respectively. Both were expensive and difficult to synthesize and were therefore not immediately considered for commercial use. In the 1980s, their development was reconsidered in light of a new appreciation that a growing proportion of anesthetic practice was taking place in the outpatient setting and that drugs halogenated exclusively with fluorine were less soluble in blood and tissues, allowing faster awakening and recovery (see Fig. 7.1 and Table 7.1 and Box 7.1 ) (also see Chapter 37 ).
Factors Increasing MAC
Drugs
Amphetamine (acute use)
Cocaine
Ephedrine
Ethanol (chronic use)
Age
Highest at age 6 months
Electrolytes
Hypernatremia
Hyperthermia
Red Hair
Factors Decreasing MAC
Drugs
Propofol
Etomidate
Barbiturates
Benzodiazepines
Ketamine
α 2 -Agonists (clonidine, dexmedetomidine)
Ethanol (acute use)
Local anesthetics
Opioids
Amphetamines (chronic use)
Lithium
Verapamil
Age
Elderly patients
Electrolyte Disturbance
Hyponatremia
Other Factors
Anemia (hemoglobin < 5 g/dL)
Hypercarbia
Hypothermia
Hypoxia
Pregnancy
MAC, Minimum alveolar concentration.
Mechanism of Action
The question of how inhaled anesthetics produce the anesthetic state may be addressed at many levels of biologic organization, including their location of action within the central nervous system, the molecules with which they interact, and the nature of this biologic interaction. Answering these questions requires an ability to measure anesthetic effects. Although inhaled anesthetics have been used to provide surgical anesthesia for almost 160 years, there is no single, accepted definition of what constitutes the anesthetic state. For experimental purposes, an operational definition of immobility in response to surgical stimulation and amnesia for intraoperative events has proved useful.
Measurable Characteristics
Measurable and universal characteristics of all inhaled anesthetics include production of immobility and amnestic effects . Immobility is measured by the minimum alveolar concentration (MAC) of anesthetic required to suppress movement to a surgical incision in 50% of patients (see Box 7.1 ). However, the presence of amnesia or awareness is difficult to assure (also see Chapter 20, Chapter 47 ). Although analgesia is part of the anesthetic state, it also cannot be measured in an immobile patient who cannot remember. Surrogate measures of pain (i.e., increased heart rate or systemic arterial blood pressure) suggest that inhaled anesthetics do not suppress the perception of painful stimuli. Some inhaled anesthetics have hyperalgesic (pain-enhancing) effects in small concentrations. Skeletal muscle relaxation is a common, but not universal, central effect of inhaled anesthetics, as evidenced by nitrous oxide, which increases skeletal muscle tone.
Immobility
Potent inhaled anesthetics produce immobility mostly by their actions on the spinal cord, as evidenced by determination of MAC in decerebrate animals. Studies in rodents suggest that nitrous oxide activates descending noradrenergic pathways originating in the periaqueductal gray matter brainstem, which in turn inhibit nociceptive input in the dorsal horn of the spinal cord.
Amnestic Effects (Also See Chapter 47 )
Supraspinal structures such as the amygdala, hippocampus, and cortex are considered highly probable targets for the amnestic effects of anesthetics.
Central Nervous System Depression and Ion Channels
Inhaled anesthetics produce central nervous system depression by their actions on ion channels, which govern the electrical behavior of the nervous system. Inhaled anesthetics probably produce anesthesia by enhancing the function of inhibitory ion channels and by blocking the function of excitatory ion channels. Enhancing the function of inhibitory ion channels leads to hyperpolarization of the neuron. Hyperpolarization results when chloride anions enter neurons through γ-aminobutyric acid A (GABA A ) receptors or glycine receptors or when there is an efflux of potassium cations out of neurons through potassium ion channels. Blocking the function of excitatory ion channels prevents depolarization of the neuron by preventing the passage of positive charges into the neuron (i.e., passage of sodium ions through N -methyl- d- aspartate [NMDA] receptors or sodium channels). Anesthetics may also affect the release of neurotransmitters, and this effect may be mediated in part by ion channels that regulate the release of neurotransmitters.
Physical Properties
Molecular Structure
Modern inhaled anesthetics, with the exception of nitrous oxide, are halogenated hydrocarbons (see Figs. 7.2 and 7.3 ). Halothane lacks the ether moiety present on isoflurane, sevoflurane, and desflurane, accounting for its capability to produce ventricular cardiac dysrhythmias. Isoflurane and desflurane differ only by the substitution of one chlorine atom for fluorine. Fluorine substitution confers greater stability and resistance to metabolism.
Vapor Pressure and Delivery
Nitrous oxide exists as a gas at ambient temperature, although it becomes a liquid at higher pressures. The remaining inhaled anesthetics are liquids at ambient temperatures.
Variable-Bypass Vaporizers (Also See Chapter 15 )
Halothane, sevoflurane, and isoflurane are delivered by variable-bypass vaporizers (Tec 4, 5, and 7; North American Draeger 19.n and 20.n). The variable-bypass vaporizer contains two streams of inflowing fresh gas—one contacting a reservoir (sump) of liquid anesthetic and the other bypassing the sump. The stream of gas traversing the sump becomes saturated with anesthetic as governed by the anesthetic’s saturated vapor pressure. Because the volatile anesthetics produce clinically useful anesthesia at partial pressures far below that of their saturated vapor pressure, the gas exiting the sump must be diluted by gas that has not come into contact with anesthetic. The concentration of anesthetic in the gas exiting the vaporizer is determined by the relative flow (i.e., splitting ratio) of fresh gas through the sump channel versus the bypass channel. Control of anesthetic output concentration exiting the vaporizer occurs when the clinician adjusts the vaporizer dial or electronic control. Variable-bypass vaporizers are temperature compensated, maintaining constant output over a wide range of temperatures, and are calibrated for each individual anesthetic according to its differing vapor pressure (see Table 7.1 ). Tilting or overfilling of a vaporizer can potentially lead to delivery of an overdose of anesthetic if anesthetic vapor gets into the bypass channel.
The Datex-Ohmeda Aladin Cassette Vaporizer, used in the Datex-Ohmeda Anesthesia Delivery Unit (ADU) machines, is a single electronically controlled vaporizer with its bypass housed within the ADU and the sump located within interchangeable, magnetically coded cassettes for delivery of halothane, enflurane, isoflurane, sevoflurane, and desflurane. The Aladin utilizes variable bypass as a means of regulating output concentration, doing so via activity of a central processing unit (CPU). The CPU receives input from multiple sources, including the concentration setting, flowmeters, and internal pressure and temperature sensors, and in turn regulates a flow control valve at the outlet of the vaporizing chamber. If pressure in the cassette (sump) exceeds that in the bypass chamber, which would occur if room temperature exceeds 22.8° C during desflurane administration, a one-way check valve is designed to close, preventing retrograde flow of anesthetic saturated gas back into the ADU with subsequent anesthetic overdose.
Heated Vaporizer
The vapor pressure of desflurane at sea level is 700 mm Hg at 20° C (near boiling state at room temperature), and delivery by a variable-bypass vaporizer can produce unpredictable concentrations. For this reason, a specially designed vaporizer (Tec 6, Datex-Ohmeda) that heats desflurane gas to 2 atm of pressure is used to accurately meter and deliver desflurane vapor corresponding to adjustments of the concentration dial by the anesthesia provider. In contrast to the variable-bypass vaporizers, the output concentration of desflurane from the Tec 6 is constant across a range of barometric pressures. Therefore, at high altitudes, the partial pressure of desflurane will be lower at a given Tec 6 vaporizer setting and output (volume percent) concentration than at sea level, leading to underdosing of the anesthetic unless an adjustment is made accounting for the higher altitude: required vaporizer setting = (desired vaporizer setting at sea level × 760 mm Hg)/local barometric pressure (in mm Hg). The converse (a greater output) can occur with variable-bypass vaporizers. However, the pharmacologically relevant quantitative parameter for anesthetic activity is partial pressure, not volume percent. Therefore, although a larger output of anesthesia from a vaporizer occurs at higher altitude for the same vaporizer setting, the delivered partial pressure, and anesthetic impact, will be similar in both locations as related to the vaporizer setting.
Economic and Environmental Considerations
Fresh gas flow rate directly impacts the quantity of volatile liquid used, and consequently the cost of the anesthetic delivery. Higher fresh gas flows (at or above minute ventilation) minimize rebreathing and allow faster equilibration between inspired and central nervous system (CNS) partial pressures. However, use of nonrebreathing flows involve loss of anesthetic to the environment and should be used only for a limited period of minutes, usually at anesthetic induction, or in the circumstance of light sedation and imminent surgical stimulation. There is growing awareness and concern regarding the contribution of inhaled anesthetic release to overall greenhouse gas emission and climate change. Potential environmental impact appears to stem from the atmospheric lifetime gas, as well as unique infrared absorption spectrum, of each anesthetic. Atmospheric longevities of inhaled anesthetics differ substantially (nitrous oxide, desflurane, sevoflurane, and isoflurane having 114, 10, 3.6, and 1.2 estimated years, respectively). Individual infrared absorption spectra differ, with desflurane relatively possessing the greatest carbon dioxide equivalent impact when compared to sevoflurane with the lowest. Although impact of inhaled anesthetics to overall climate change remains a topic of controversy, several points warrant consideration. First, use of low fresh gas flows (0.5-1 L/min) will offset cost and release to the environment. Second, development of systems to reclaim and reuse anesthetics hold promise to further limit environmental impact and save money.
Stability
Anesthetic degradation by metabolism or by an interaction with carbon dioxide absorbents (especially when desiccated) produces several potentially toxic compounds.
Metabolism and Degradation
Methoxyflurane produces inorganic fluoride, which was responsible for the sporadic incidence of nephrotoxicity (i.e., high-output renal failure) after prolonged anesthesia in the past. Compound A (i.e., fluoromethyl-2,2-difluoro-1-[trifluoromethyl] vinyl ether), produced from the breakdown of sevoflurane, and a similar compound produced from halothane are nephrotoxic in animals after prolonged exposure. In humans, prolonged anesthesia with sevoflurane and low fresh gas flows (1 L/min) results in compound A exposure adequate to produce transient proteinuria, enzymuria, and glycosuria, but there is no evidence of increased serum creatinine concentrations or long-term deleterious effects on renal function. Nevertheless, the package insert for sevoflurane recommends low fresh gas flow (<2 L/min) be restricted to less than 2 MAC hours (i.e., MAC concentration × duration of administration) of sevoflurane anesthesia.
Carbon Dioxide Absorbents and Exothermic Reactions
Variables influencing the amount of volatile anesthetic degradation on exposure to carbon dioxide absorbents include the condition (i.e., hydration and temperature) and chemical makeup of the absorbent, fresh gas flow rates, minute ventilation, and, most important, the anesthetic itself. Although desflurane and isoflurane are very stable in hydrated carbon dioxide absorbents up to temperatures of more than 60° C, full desiccation of conventional carbon dioxide absorbents containing sodium and potassium hydroxide causes degradation and carbon monoxide production from all volatile anesthetics regardless of temperatures (see Table 7.1 ). High fresh gas flow rates (especially those exceeding normal minute ventilation) accelerate the desiccation of absorbent, and the desiccation leads to accelerated degradation. Because degradation is an exothermic process, the absorbent temperature may increase dramatically.
The exothermic reaction that results from interaction of desiccated carbon dioxide absorbent and volatile anesthetics (especially sevoflurane) can produce extremely high temperatures inside the absorbent canister. The temperature increase may lead to explosion and fire in the canister or anesthetic circuit. The remote risk of fire and explosion from exothermic reactions can be avoided entirely by employing measures that ensure maintenance of adequate hydration in the carbon dioxide absorbent (i.e., changing the absorbent regularly, turning fresh gas flow down or off on unattended anesthesia machines, limiting fresh gas flow rates during anesthesia, and when in doubt about the hydration of the absorbent, changing it). Commercially available carbon dioxide absorbents with decreased or absent monovalent bases (i.e., sodium hydroxide and potassium hydroxide) do not undergo extensive degradation on exposure to volatile anesthetics, regardless of the absorbent hydration status.
Relative Potency of Inhaled Anesthetics
The relative potency between inhaled anesthetics is most commonly described by the dose required to suppress movement in 50% of patients in response to surgical incision. This dose (a single point on a dose-response curve) is designated the MAC. Because the standard deviation in the MAC is approximately 10%, 95% of patients should not move in response to incision at 1.2 MAC of the inhaled anesthetic, and 99% of patients should not move in response to incision at 1.3 MAC of the inhaled anesthetic. MAC is affected by several variables but is unaffected by gender or duration of surgery and anesthesia (see Box 7.1 ).
MAC allows potencies to be compared among anesthetics (see Table 7.1 ); 1.15% isoflurane is equipotent with 6% desflurane in preventing movement in response to a surgical incision in patients of a similar age and body temperature. Remarkably, MAC values for different inhaled anesthetics are additive. For example, 0.5 MAC of nitrous oxide administered with 0.5 MAC of isoflurane has the same effect as 1 MAC of any inhaled anesthetic in preventing movement in response to incision (reflecting anesthetic-induced inhibition of reflex responses at the level of the spinal cord). The concentration of anesthetic at the brain needed to prevent movement in response to a surgical incision is likely to be larger than the MAC.
The anesthetic dose required to produce amnesia probably has more variability than the MAC. The alveolar concentration of isoflurane preventing recall of a verbal stimulus was 0.20 MAC in 50% and 0.40 MAC in 95% of volunteers. Assuming a standard normal distribution of dose response, the standard deviation in minimum concentration preventing recall is therefore approximately half the mean value (0.1 MAC). Referring to standard normal curves, we can calculate that the concentration needed by 1 in 100,000 subjects with the highest anesthetic requirement would be 4.27 standard deviations (SD) above the mean (i.e., greater than 0.627 MAC) to prevent recall of verbal stimulus. Extrapolation of this value to the context of surgery must be made with caution, however, because the dose required to prevent recall of painful as opposed to verbal stimulation may be considerably larger. The ratio of concentration needed to prevent motor response to surgical incision (reflected in MAC) to that required to suppress consciousness and prevent recall differs slightly between individual potent inhaled anesthetics and differs substantially between potent inhaled anesthetics collectively versus nitrous oxide. Volunteers given isoflurane did not exhibit recall given 0.45 MAC of isoflurane, whereas recall did occur with as much as 0.6 MAC of nitrous oxide.
Pharmacokinetics of Inhaled Anesthetics
Pharmacokinetics of inhaled anesthetics describes their uptake (absorption) from alveoli into the systemic circulation, distribution in the body, and eventual elimination by the lungs or metabolism principally in the liver ( Box 7.2 ). By controlling the inspired partial pressure (P i ) (same as the concentration [%] when referring to the gas phase) of an inhaled anesthetic, a gradient is created such that the anesthetic is delivered from the anesthetic machine to its site of action, the brain. The primary objective of inhaled anesthesia is to achieve a constant and optimal brain partial pressure (Pbr) of the anesthetic.
Transfer of inhaled anesthetic from anesthetic machine to alveoli
Inspired partial pressure
Alveolar ventilation
Characteristics of anesthetic breathing system
Transfer of inhaled anesthetic from alveoli to arterial blood
Blood-gas partition coefficient
Cardiac output
Alveolar-to-venous partial pressure difference
Transfer of inhaled anesthetic from arterial blood to brain
Brain-blood partition coefficient
Cerebral blood flow
Arterial-to-venous partial pressure difference
The brain and all other tissues equilibrate with the partial pressure of the inhaled anesthetic delivered to them by the arterial blood (Pa). Likewise, the blood equilibrates with the alveolar partial pressure (P a ) of the anesthetic:
PA⇄Pa⇄Pbr
Maintaining a constant and optimal P a becomes an indirect but useful method for controlling the Pbr. The P a of an inhaled anesthetic mirrors its Pbr and is the reason the P a is used as an index of anesthetic depth, a reflection of the rate of induction and recovery from anesthesia, and a measure of equal potency (see earlier discussion under “Relative Potency of Inhaled Anesthetics”). Understanding the factors that determine the P a and the Pbr allows the anesthesia provider to skillfully control and adjust the dose of inhaled anesthetic delivered to the brain.
Factors That Determine the Alveolar Partial Pressure
The P a and ultimately the Pbr of an inhaled anesthetic are determined by input (delivery) into the alveoli minus uptake (loss) of the drug from the alveoli into the pulmonary arterial blood. Input of the inhaled anesthetic depends on the P i , alveolar ventilation ( <SPAN role=presentation tabIndex=0 id=MathJax-Element-2-Frame class=MathJax style="POSITION: relative" data-mathml='V˙A’>V˙AV˙A
V ˙ A
), and characteristics of the anesthetic breathing system. Uptake of the inhaled anesthetic depends on the solubility, cardiac output (CO), and alveolar-to-venous partial pressure difference (P a − Pv). These six factors act simultaneously to determine the P a . Metabolism and percutaneous loss of inhaled anesthetics do not significantly influence P a during induction and maintenance of anesthesia.
Inspired Anesthetic Partial Pressure
A high P i is necessary during initial administration of an inhaled anesthetic. This initial high P i (i.e., input) offsets the impact of uptake into the blood and accelerates induction of anesthesia as reflected by the rate of increase in the P a . This effect of the P i is known as the concentration effect. Clinically, the range of concentrations necessary to produce a concentration effect is probably possible only with nitrous oxide ( Fig. 7.4 ).

With time, as uptake into the blood decreases, the P i should be decreased to match the decreased anesthetic uptake. Decreasing the P i to match decreasing uptake with time is critical if the anesthesia provider is to achieve the goal of maintaining a constant and optimal Pbr. For example, if the P i were maintained constant with time (input constant), the P a (and depth of anesthesia as reflected by the Pbr) would progressively increase as uptake of the anesthetic into the blood diminished with time.
Second Gas Effect
The second gas effect is a distinct phenomenon that occurs independently of the concentration effect. The ability of the large-volume uptake of one gas (first gas) to accelerate the rate of increase of the P a of a concurrently administered companion gas (second gas) is known as the second gas effect. For example, the initial large volume uptake of nitrous oxide accelerates the uptake of companion gases such as volatile anesthetics and oxygen. The transient increase (about 10%) in Pa o 2 that accompanies the early phase of nitrous oxide administration reflects the second gas effect of nitrous oxide on oxygen. This increase in Pa o 2 has been designated alveolar hyperoxygenation. Increased tracheal inflow of all inhaled gases (i.e., first and second gases) and concentration of the second gases in a smaller lung volume (i.e., concentrating effect) because of the high-volume uptake of the first gas are the explanations for the second gas effect. Although the second gas effect is based on proven pharmacokinetic principles, its clinical importance is doubtful.
Alveolar Ventilation
Increased <SPAN role=presentation tabIndex=0 id=MathJax-Element-3-Frame class=MathJax style="POSITION: relative" data-mathml='V˙A’>V˙AV˙A
V ˙ A
, like P i , promotes input of inhaled anesthetics to offset uptake into the blood. The net effect is a more rapid rate of increase in the P a and induction of anesthesia. Predictably, hypoventilation has the opposite effect, acting to slow the induction of anesthesia.
Controlled ventilation of the lungs that results in hyperventilation and decreased venous return accelerates the rate of increase of the P a by virtue of increased input (i.e., increased <SPAN role=presentation tabIndex=0 id=MathJax-Element-4-Frame class=MathJax style="POSITION: relative" data-mathml='V˙A’>V˙AV˙A
V ˙ A
) and decreased uptake (i.e., decreased CO). As a result, the risk of anesthetic overdose may be increased during controlled ventilation of the lungs, and it may be appropriate to decrease the P i of volatile anesthetics when ventilation of the lungs is changed from spontaneous to controlled to maintain the P a similar to that present during spontaneous ventilation.
Another effect of hyperventilation is decreased cerebral blood flow because of the associated decrease in the Pa co 2 . Conceivably, the impact of increased anesthetic input on the rate of increase of the P a would be offset by decreased delivery of anesthetic to the brain. Theoretically, coronary blood flow may remain unchanged, such that increased anesthetic input produces myocardial depression, and decreased cerebral blood flow prevents a concomitant onset of central nervous system depression.
Anesthetic Breathing System (Also See Chapter 15 )
Characteristics of the anesthetic breathing system that influence the rate of increase of the P a include the volume of the system, solubility of inhaled anesthetics in the rubber or plastic components of the system, and gas inflow from the anesthetic machine. The volume of the anesthetic breathing system acts as a buffer to slow attainment of the P a . High gas inflow from the anesthetic machine negates this buffer effect. Solubility of inhaled anesthetics in the components of the anesthetic breathing system initially slows the rate at which the P a increases. At the conclusion of an anesthetic, reversal of the partial pressure gradient in the anesthetic breathing system results in elution of the anesthetics that slows the rate at which the P a decreases.
Solubility
The solubility of inhaled anesthetics in blood and tissues is denoted by partition coefficients (see Table 7.1 ). A partition coefficient is a distribution ratio describing how the inhaled anesthetic distributes itself between two phases at equilibrium (when the partial pressures are identical). For example, a blood-gas partition coefficient of 10 means that the concentration of the inhaled anesthetic is 10 in the blood and 1 in the alveolar gas when the partial pressures of that anesthetic in these two phases are identical. Partition coefficients are temperature dependent. For example, the solubility of a gas in a liquid is increased when the temperature of the liquid decreases. Unless otherwise stated, partition coefficients are given for 37° C.
Blood-Gas Partition Coefficient
High blood solubility means that a large amount of inhaled anesthetic must be dissolved (i.e., undergo uptake) in the blood before equilibrium with the gas phase is reached. The blood can be considered a pharmacologically inactive reservoir, the size of which is determined by the solubility of the anesthetic in the blood. When the blood-gas partition coefficient is high, a large amount of anesthetic must be dissolved in the blood before the Pa equilibrates with the P a ( Fig. 7.5 ). Clinically, the impact of high blood solubility on the rate of increase of the P a can be offset to some extent by increasing the P i . When blood solubility is low, minimal amounts of the anesthetic have to be dissolved in the blood before equilibrium is reached such that the rate of increase of the P a and that of the Pa and Pbr are rapid (see Fig. 7.5 ).
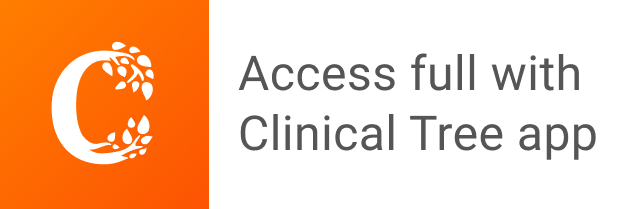