FIG. 20.1 Signs and reflex reactions of stages of anesthesia. (Adapted from Gillespie NA: Signs of anesthesia, Anesth Analg 22:275, 1943.)
Most surgical procedures in which ether anesthesia was used were performed at anesthesia stage III, which is divided into four planes.4 Plane 1 is entered when the lid reflex is abolished and respiration becomes regular. During this plane, the vomiting reflex is gradually abolished. The nurse working in the PACU must know that swallowing, retching, and vomiting reflexes tend to disappear in that order during induction and reappear in the same order during emergence from anesthesia.
Plane 2 lasts from the time the eyeballs cease to move and become concentrically fixed to the beginning of a decrease of activity of the intercostal muscles or thoracic respiration. The reflex of laryngospasm disappears during this plane. Plane 3 is entered when intercostal activity begins to decrease. Complete intercostal paralysis occurs in lower plane 3, and respiration is produced solely by the diaphragm. Plane 4 lasts from the time of paralysis of the intercostal muscles to the cessation of spontaneous respiration.
Tracheal tug often appears in association with deep anesthesia and intercostal paralysis. This effect represents an unopposed action of the diaphragm, which displaces the hilum of the lung and thereby increases traction on the trachea.
Stage IV lasts from the time of cessation of respiration to failure of the circulatory system. This level of anesthesia is called the stage of overdose.
When ether was used as the sole inhalation agent, these signs and stages were seen in reverse order on emergence from the anesthetic. No single clinical sign can be considered a reliable indicator of anesthetic depth by itself. All clinical signs must be viewed in the context of the patient’s status along with the particular characteristics of the individual anesthetic agent used.
Some of the more reliable indicators of depth of anesthesia for the more modern inhalation anesthetics include changes in breathing pattern, eye movement, lacrimation, and muscle tone. Because ventilation is under autonomic control, it is the most sensitive indicator of depth of anesthesia. In the PACU, a patient who uses diaphragmatic ventilation without the intercostal muscles should be considered to be in surgical anesthesia. As the ventilatory pattern returns to a more normal rate, rhythm, and pattern, the patient can be considered to be in light anesthesia and about to have total emergence. Eye movement as opposed to pupillary size is a good indicator of anesthetic depth. Light anesthesia is present with eye movement. Deeper anesthesia is present when the eyes are close together in a cross-eyed position. Lacrimation does not occur during surgical anesthesia when a patient receives desflurane (Suprane), isoflurane (Forane), or sevoflurane (Ultane). Conversely, if a patient received one of those drugs and has tearing, light anesthesia can be considered to be present. As the depth of anesthesia is increased, the amount of muscle tone decreases. Therefore, if a patient in the PACU lacks muscle tone, especially in the jaw and abdomen, the patient should be considered to be in a surgical depth of anesthesia. With the assessment of the degree of muscle tone, the perianesthesia nurse must critically assess the degree of reversal of skeletal muscle relaxants (see Chapter 23) before determining the depth of anesthesia with the criterion of muscle tone. Finally, because the determinants of anesthesia depth have such a high degree of variability, all possible assessment tools should be incorporated into the care of the patient in the PACU. The bottom line is constant vigilance of the patient’s physiologic parameters during emergence from anesthesia and the institution of appropriate nursing interventions based on an ongoing assessment.
Pharmacokinetics of Inhalation Anesthetics
The pharmacokinetics of inhalation anesthetics involve uptake, distribution, metabolism, and elimination. Basically, this involves a series of partial pressure gradients starting in the anesthesia machine to the patient’s brain for induction, and vice versa for emergence. The object of anesthesia is a constant and optimal partial pressure in the brain. The key to attainment of anesthesia is the alveolar partial pressure (PA) in equilibrium with the arterial partial pressure (Pa) and brain partial pressure (Pbr) of the inhaled anesthetic.4 The partial pressure of an inhalation anesthetic in the brain is used to determine the depth of anesthesia. The more potent the anesthetic, the lower the partial pressure of the agent needed to produce a certain depth of anesthesia.
Movement of Inhalation Anesthetic From Anesthesia Machine to Alveoli
The determinants of the PA are the inspired partial pressure of the inhalation anesthetic, the characteristics of the anesthesia machine’s delivery system, and the patient’s alveolar ventilation. The inhaled partial pressure (PI) is the concentration of the inhalation anesthetic delivered from the anesthesia machine. The effect of the PI on the rate of increase in the PA is called the concentration effect. The higher the inhaled concentration, the more rapid the induction of anesthesia. The anesthesia machine’s delivery system has an effect on the depth of anesthesia and the speed of induction and emergence. For example, the rate of uptake of an anesthetic agent administered via inhalation can be reduced with the diffusion of the anesthetic agent into the rubber tubing of the anesthesia machine, the small losses of anesthetic agent from the body via diffusion across skin and mucous membranes, and, to a lesser extent, the metabolism of the agents by the body.
Alveolar ventilation plays the primary role in delivery of the anesthetic gas. It is determined in large part by the minute ventilation (V˙E
). If the V˙E
is high, the anesthetic concentration increases quickly in the alveoli as does the concentration in the arterial blood. This concept is important to understand because the reverse also is true. In the emergence phase of anesthesia, a good V˙E
is important to ensure elimination of the anesthetic agent.



Movement of Inhalation Anesthetic From Alveoli to Arterial Blood
The movement of the inhalation anesthetic agent from the alveoli to the arterial blood depends on the blood-gas partition coefficient and the cardiac output. The rate at which the anesthetic is taken up by the blood and tissues is governed in part by the solubility of the agent in blood. This is expressed as the blood-gas partition coefficient, or the Oswald solubility coefficient, and is defined as the ratio of the concentration of an anesthetic in blood to that in a gas phase when the two are in equilibrium (Table 20.2). This concept is difficult to understand because the more soluble the anesthetic agent is, the slower the agent is in producing anesthesia. This effect is because the blood serves as a reservoir, and a large volume of the agent must be introduced to attain an equilibrium between the blood partial pressure and the partial pressure in the lungs.
The blood conveys the anesthetic agent to the tissues. Consequently, a normal cardiac output is needed for facilitation of the movement of the inhalation anesthetic through the tissues to the brain. The partial pressure increases most rapidly in the tissues with the highest rates of blood flow. Of interest is the great variation in blood perfusion of certain tissues in the body. The body tissue compartments can be divided into the following major groups:
• The vessel-rich group, which consists of the heart, brain, kidneys, hepatoportal system, and endocrine glands
• The intermediate group of perfused tissues, which consists of muscle and skin
• The fat group, which includes marrow and adipose tissue
• The vessel-poor group, which has the poorest circulation per unit volume and comprises tendons, ligaments, connective tissue, teeth, bone, and other avascular tissue
The vessel-rich group of tissues receives 75% of the cardiac output; thus, the brain becomes saturated rapidly with an anesthetic agent administered via inhalation. On termination of the anesthetic, the reverse takes place, and the agent is rapidly removed from the brain.
The tissue tensions of the inhaled anesthetic increase and approach the arterial blood tension and ultimately the PA. One of the tissue groups that affect both the induction and the emergence from anesthesia is the fat group. The oil-gas partition coefficient best exemplifies the process involved with the affinity of anesthesia agents to adipose tissue and ultimately the emergence from anesthesia. The oil-gas partition coefficient is defined as the ratio of the concentration of the anesthetic agent in oil (adipose tissue) to that in a gaseous phase when the two are in equilibrium (see Table 20.2). The oil-gas partition coefficients seem to parallel anesthetic requirements. In fact, it is possible to calculate the MAC by knowing the oil-gas partition coefficient. With the constant of 150, the calculated MAC for an anesthetic with an oil-gas partition coefficient of 100 is 1.5%.
Because some anesthetic agents are highly fat soluble, they tend to be readily absorbed by the adipose tissue. This characteristic affects uptake of the anesthetic agent, but of more importance is the prolonged recovery phase that usually ensues with a high oil-gas partition coefficient such as in the case of halothane. Because adipose tissue is poorly perfused by blood, the adipose tissue releases the agent slowly to the blood at the termination of the anesthesia. Redistribution then takes place; some of the agent is eliminated by the lungs, which are vessel rich, and some is distributed to the brain. The recovery period becomes significantly extended when the administration time of the anesthetic agent is prolonged to allow for complete saturation of the adipose tissue.
Halothane (Fluothane) was the classic inhalation anesthetic from the 1960s to the 1990s. Inclusion of halothane in the discussion is helpful because all the inhalation anesthetic agents currently in use have almost the same partition coefficients. Halothane has an oil-gas partition coefficient approximately twice that of isoflurane, desflurane, or sevoflurane; therefore, with use of halothane as the marker, the newer inhalation agents are twice as fast during induction and emergence as before. All three of the current inhalational anesthetics are used in a variety of settings, and all possess a blood-gas partition coefficient less than 1 (see Table 20.2).
Movement of Inhalation Anesthetic From Arterial Blood to the Brain
The transfer of the inhalation anesthetic from the arterial blood to the brain depends on the blood-brain partition coefficient of the agent and the cerebral blood flow. The blood-brain partition coefficient for most of the inhalation anesthetics is between 1.3 and 2 (see Table 20.2). The concentration gradient during induction of anesthesia is as follows:
PA>Pa>Pbr
During maintenance of surgical anesthesia, the brain tissue becomes saturated with the anesthetic agent, and the brain tissue is in equilibrium with the alveolar and arterial concentration. Consequently:
PA=Pa=Pbr
Emergence of Inhalation Anesthesia
When the administration of the anesthetic is terminated, a reverse gradient takes place. In this instance, the PA is almost zero because only oxygen is administered during the emergence phase. The gradient that develops is as follows:
PA<Pa<Pbr
This gradient favors the removal of the anesthetic agent from the brain tissue. The partial pressure in the tissues declines first and is followed by that in the arterial blood. The agent returns to the lungs and is then eliminated into the atmosphere. The factors that affect the rate of elimination of the agent are the same ones that determine how rapidly an anesthetic agent takes a patient to surgical anesthesia. If a short procedure is performed (less than 1 hour), complete equilibrium among PA, Pa, and Pbr might not have occurred, and the recovery from anesthesia is more rapid.4 The reverse is true; during long procedures in which equilibrium occurs, a prolonged emergence may be anticipated.
Potency of Inhalation Anesthetic Agents
Potency is determined by factors such as absorption, distribution, metabolism, excretion, and affinity for a receptor. The potency of the anesthetic agent refers to its ability to take the patient through all the stages of anesthesia to respiratory and circulatory arrest without the occurrence of hypoxia or the use of preanesthetic medication. Certainly, circulatory and respiratory arrests are not desired outcomes of the use of anesthetic agents; these features are used merely to describe the potency of clinically used anesthetic agents. For example, isoflurane is 100% potent compared with nitrous oxide, which is 15% potent. Isoflurane, when administered with oxygen to meet the patient’s metabolic needs and when given without premedication, takes the patient to circulatory and respiratory arrest, whereas nitrous oxide administered with oxygen takes the patient only to the first portion of surgical anesthesia and no further. Therefore, clinically speaking, the potency of a drug makes little difference as long as the drug to be administered has an ED for a particular patient, which is why the concept of ED was developed. The ED is the dose of a drug necessary to produce certain effects in a certain percentage of patients. For example, an ED50 means that a drug produces a particular effect in 50% of the patients.4
Another method of determination of potency or strength is with the use of the MAC. The MAC is found by determining the alveolar concentration (at 1 atm) needed for prevention of gross muscular movement in response to painful stimuli in 50% of anesthetized patients. The MAC is actually a median value and not a minimum value as the term appears to imply. The lower the MAC value, the higher the anesthetic potency of the inhalation anesthetic agent. Consequently, the MAC defines the therapeutic effect of inhalational anesthetics as the prevention of movement in response to surgical stimulation.4
The potency of an inhalation anesthetic agent with the MAC as the tool of assessment varies with the patient’s age, state of health, clinical conditions, and concurrent use of other drugs (with depressant effects). The factors that modify the MAC are presented in Box 20.1. MAC-Awake is the anesthetic dose at which 50% of the patients respond to the command “open your eyes”; it is also the dose of anesthetic at which most patients lose consciousness and recall. MAC-Awake usually corresponds with stage I of anesthesia. A more scientific method can be used to address potency of the inhalational agents—the MAC-Awake value is divided by the MAC, which is referred to as the MAC-Awake/MAC ratio. In this case, the inhalational agents such as desflurane, sevoflurane, and isoflurane have ratios between 0.3 and 0.4 and are therefore considered 100% potent agents, whereas nitrous oxide has a ratio of 0.64 and is therefore considered a weak amnestic agent.2 Another term used with MAC is MAC-Block adrenergic response, or MAC-BAR, which is the MAC needed to block the adrenergic and cardiovascular responses to incision; it corresponds to stage III, plane 3 anesthesia. Adrenergic responses to a skin incision, such as heart rate, rate-pressure product, and mean arterial pressure, can be used. Table 20.2 presents the MAC values for the inhalational anesthetic agents, and Box 20.1 describes the factors that reduce, increase, or have no effect on the MAC of inhaled anesthetics.
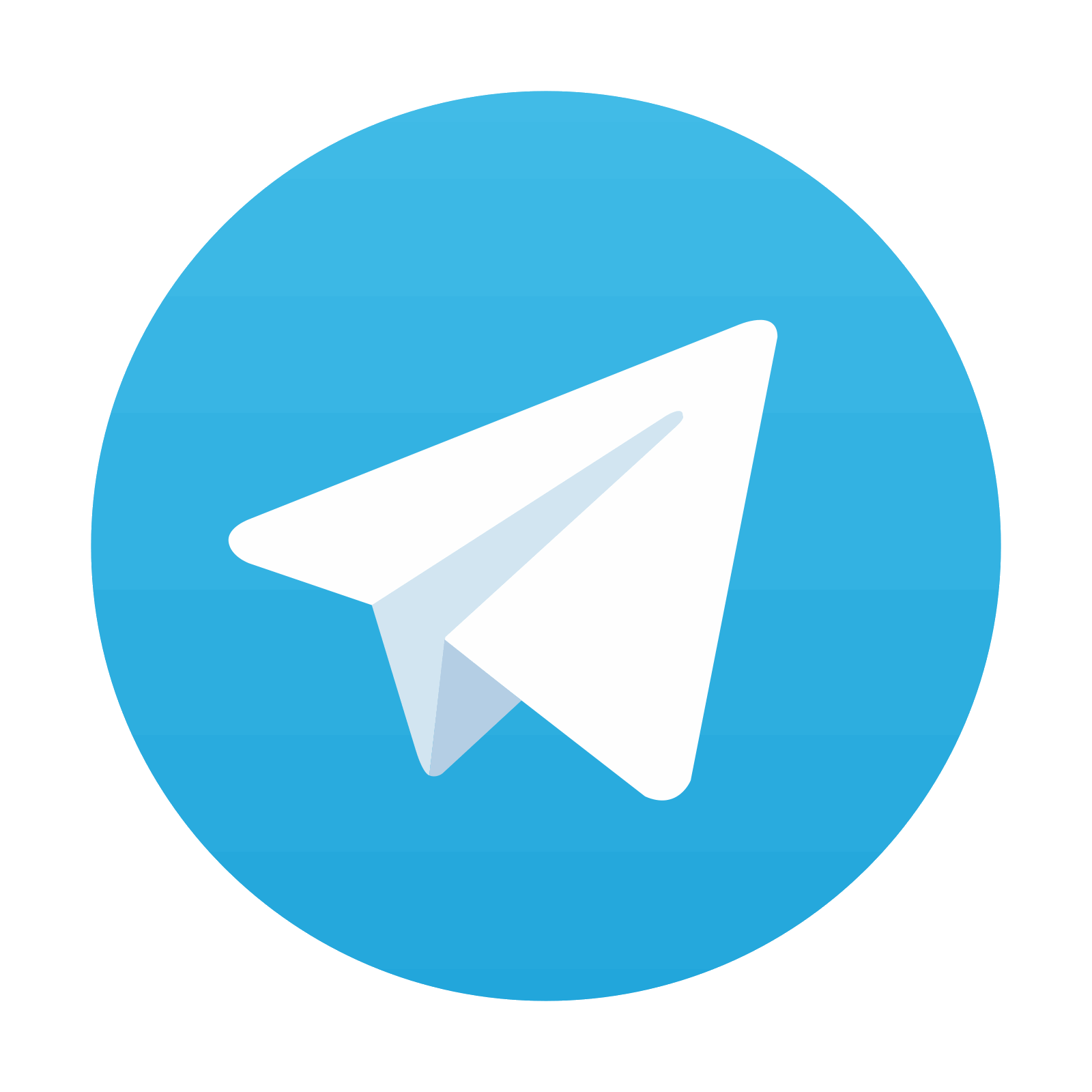
Stay updated, free articles. Join our Telegram channel
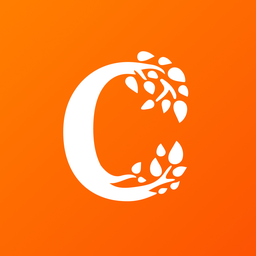
Full access? Get Clinical Tree
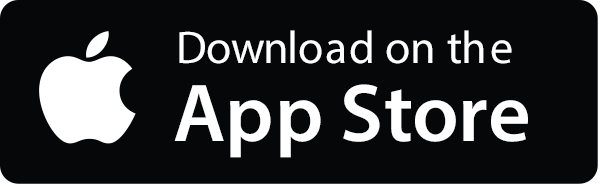
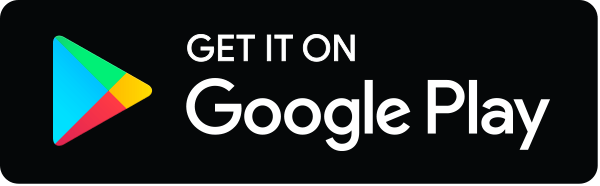