In Utero Fetal Surgery and Ex Utero Intrapartum Therapy (EXIT)
David G. Mann
Olutoyin A. Olutoye
Introduction
The majority of prenatally diagnosed fetal conditions are amenable to medical or surgical intervention following delivery. However, there are a few that benefit from interventions during pregnancy. For conditions that may benefit from fetal intervention, the basic framework of understanding includes (a) the natural history of the untreated disease “in utero” must be documented before clinical application of the fetal procedure, (b) there must be sound pathophysiologic rationale to develop a prenatal treatment strategy, (c) there is a demonstration of safety and efficacy of the fetal procedure in an appropriate animal model, and (d) inclusion and exclusion selection criteria have been developed for treatment (1).
In utero fetal surgery can be performed via a percutaneous approach or through a uterine incision (hysterotomy). Percutaneous procedures are usually ultrasound guided and include intrauterine transfusions and shunt placements. In addition, fetoscopic procedures for laser photocoagulation of abnormal placental anastomoses, or placement of tracheal plugs for tracheal occlusion in congenital diaphragmatic hernia (CDH), can also be performed percutaneously.
Procedures performed via a hysterotomy or uterine incision are of two types: Open fetal surgery in which surgery is performed during pregnancy on mid-gestation fetuses and the pregnancy is allowed to continue to near term, and ex utero intrapartum therapy (EXIT) in which a procedure or surgery is performed on fetuses just prior to delivery.
This chapter will describe the indications for fetal intervention via the percutaneous and open approach as well as the anesthetic management for these different procedures.
Percutaneous Fetal Interventions
A variety of procedures are performed percutaneously. Some require fetal analgesia while other procedures, which do not involve the fetus directly, may not warrant direct administration of medication to the fetus. Specific discussion of fetal medications will be addressed where indicated. Maternal anesthesia and/or analgesia required for percutaneous interventions will be discussed at the end of the description of the various procedures.
Intrauterine Transfusions
Hemolytic disease of the newborn, mostly the result of Rhesus alloimmunization, was previously a significant cause of perinatal mortality. However, elucidation of the pathophysiology, development of diagnostic tools, and effective prophylaxis has reduced its occurrence to a rarity (2). Red-cell alloimmunization may occur when a blood-type incompatibility (Rh-D or Kell) exists between mother and fetus. Fetal red blood cells that cross the placenta may stimulate the production of maternal antibodies resulting in red blood cell hemolysis in the fetus, and fetal anemia. While Rh-D alloimmunization is the most common etiology of fetal anemia, Kell alloimmunization, severe fetomaternal hemorrhage, placental chorioangiomas, homozygous α-thalassemia, and parvovirus B19 can also cause fetal anemia that may require fetal blood transfusion (2).
The incidence of rhesus D (Rh-D) alloimmunization is approximately 6/1,000 live births. As this is a rare entity, experts suggest that affected patients should be referred to a maternal–fetal medicine specialist experienced in treating red-cell alloimmunization in pregnancy, as some patients will require treatment with an intrauterine fetal transfusion (3). Intrauterine transfusion involves insertion of a needle through the maternal abdominal wall, uterus, and into a fetal vein (umbilical or intrahepatic approach) under ultrasound guidance. Transfusion may also be via the intraperitoneal or intracardiac approach although the intracardiac approach is usually utilized as a last resort.
For these procedures, drugs are administered to the fetus following maternal sedation or local anesthetic infiltration into the maternal abdomen. Fetal immobility is important for this procedure as fetal movement may result in laceration of the vessel at the puncture site with hemorrhage into the amniotic cavity particularly when transfusion is via a fetal intravascular approach (2). In order to prevent fetal movement, a neuromuscular blocking drug is administered directly to the fetus. Fetal analgesia is also provided with direct administration of opioids to the fetus particularly with the intrahepatic, intraperitoneal, or intracardiac approach. Further details of fetal analgesia and immobility and maternal sedative/anesthetic requirements for percutaneous procedures are discussed later in the chapter.
Intrauterine transfusion procedures are safe, with an overall survival rate of approximately 90% and a complication rate of approximately 3% (4). Possible complications that may occur during transfusion include inadvertent uterine artery puncture leading to arterial spasm and fetal bradycardia; tamponade, hemopericardium, or arrhythmias following cardiac puncture; cord accidents causing fetal distress; volume overload, preterm rupture of membranes, or preterm labor (2). Complications that have been reported following the procedure include rupture of membranes (0.1%), intrauterine infection (0.3%), fetal and neonatal death (0.7% and 0.9%, respectively), as well as emergency cesarean section (2.0%) (5).
Shunts
Lesions characterized by fluid-filled spaces amenable to decompression via shunt placement or aspiration, may be identified by ultrasound during pregnancy. These lesions include lower urinary tract obstruction (LUTO) which may
cause an obstructive uropathy (6); congenital cystic adenomatoid malformations (CCAMs) or idiopathic pleural effusions (IPEs), both of which may cause pulmonary hypoplasia and cardiac failure from compression of mediastinal structures (6). Fetuses with any of these fluid-filled lesions may benefit from placement of shunts such as a vesicoamniotic or thoracoamniotic shunt to divert fluid from the enclosed space into the amniotic sac. These shunts are intended to halt or reverse the pathophysiologic changes resulting from obstruction of the normal fluid egress.
cause an obstructive uropathy (6); congenital cystic adenomatoid malformations (CCAMs) or idiopathic pleural effusions (IPEs), both of which may cause pulmonary hypoplasia and cardiac failure from compression of mediastinal structures (6). Fetuses with any of these fluid-filled lesions may benefit from placement of shunts such as a vesicoamniotic or thoracoamniotic shunt to divert fluid from the enclosed space into the amniotic sac. These shunts are intended to halt or reverse the pathophysiologic changes resulting from obstruction of the normal fluid egress.
Lower Urinary Tract Obstruction (LUTO)
An enlarging fluid-filled fetal bladder may result from LUTO. This occurs most commonly in male fetuses with urethral abnormalities such as posterior urethral valves or urethral atresia. However, anterior urethral valves, meatal stenosis, and a hypoplastic mid-urethra may also lead to LUTO. LUTO is less common in female fetuses in which it may occur as a result of abnormal cloacal development. This type of obstruction can also be associated with congenital syndromes such as megacystis-microcolon syndrome, megacystis-megaureter syndrome, Trisomy 21, and Trisomy 18 (6). Complete urethral obstruction can lead to bladder distention and hydroureteronephrosis, possibly causing renal fibrocystic dysplasia in the fetus. Oligohydramnios occurs as a result of decreased urine entering the amniotic space and pulmonary hypoplasia may also occur due to decreased amniotic fluid entering the fetal lungs. Secondary deformities of the face and extremities have also been noted to occur in these patients (Potter’s syndrome) (7). Postnatal morbidity and mortality depends on pulmonary development and renal function. Oligohydramnios with urethral obstruction occurring in early mid-gestation is associated with an estimated 95% mortality rate (8).
Determining prenatal renal injury in obstructive uropathy has been challenging. The prognosis for postnatal renal and pulmonary function have been separated into “good” and “poor” based on fetal urine characteristics as well as other findings such as the ultrasound appearance of fetal kidneys (9). Favorable and poor prognostic indicators based on urine values are shown in Table 49-1.
Technically, the fetal bladder must be drained at least three times with 24- to 48-hour intervals between each drain and within a 5- to 7-day period in order to establish the pattern (increasing or decreasing) of hypertonicity. The first drainage would collect urine that has been collecting in the fetal bladder for an unknown period of time and would not reflect current function. The second drainage would collect urine present in the upper renal system that drained into the bladder following the first “decompressing” drainage and still would not reflect the current function. Finally, the third drainage will collect recently formed urine that adequately represents the current underlying renal function. Increasing urinary hypertonicity is reflective of progressive or advanced renal dysfunction, depicts a “poor prognosis” for the fetus, and renders the fetus ineligible for in utero therapy. Decreasing hypertonicity is believed to reflect the potential for renal salvage, and in this situation, in utero intervention may be beneficial (10).
Table 49-1 Prognostic Urinalysis Values for Postnatal Renal and Pulmonary Function in Obstructive Uropathy | ||||||||||||||||||||||||
---|---|---|---|---|---|---|---|---|---|---|---|---|---|---|---|---|---|---|---|---|---|---|---|---|
|
For eligible cases of LUTO, in utero therapy involves insertion of a vesicoamniotic shunt that diverts urine from the obstructed fetal bladder into the amniotic sac. This temporizing therapy is intended to “decompress” the developing kidneys in order to halt and possibly reverse renal damage; hence, a neonatal procedure will likely still be necessary following delivery. Under ultrasound visualization, a shunt trocar is inserted percutaneously through the maternal abdominal wall, uterus, and into the amniotic sac near the lower fetal abdomen. The trocar tip is positioned inferolateral to the abdominal cord insertion site. Color Doppler confirms preservation of the umbilical arteries as the trocar is passed through the fetal abdominal wall and into the bladder. A shunt is deployed through the trocar with one “coiled” end positioned in the fetal bladder, a straight channel spanning the fetal abdominal wall, and the other “coiled” end placed in the amniotic sac. Shunt position and the initiation of bladder drainage are confirmed by ultrasound at the end of the procedure.
Possible complications of this procedure include chorioamnionitis, premature rupture of the fetal membranes, preterm labor, intraplacental bleeding, and iatrogenic gastroschisis. Shunts may also become dislodged with an occurrence rate of approximately 40% (6). Subsequent delivery of treated fetuses is managed by routine obstetrical indications, with vaginal delivery occurring typically at a gestational age of 34 to 35 weeks. Biard et al. (11) reported on long-term outcomes of fetuses with LUTO: The final postnatal diagnoses are most commonly posterior urethral valves (39%) and prune belly syndrome (39%). The overall 1-year survival rate is 91%, with mortality attributable to pulmonary hypoplasia. In the same review, at a median of 5.8 years, spontaneous voiding was possible in 61% of cases; renal function was “acceptable” in 44%, “mildly insufficient” in 22%; and 34% of affected fetuses’ required renal transplant. In addition, 44% had persistent respiratory problems, 66.5% experienced poor growth, and 50% had frequent urinary tract infections.
Congenital Cystic Adenomatoid Malformation (CCAM)
An enlarging fluid-filled chest mass may result from a congenital cystic adenomatoid malformation or CCAM. The pathophysiology of CCAM is discussed in more detail later in this chapter. Thoracoamniotic shunt placement may be indicated for CCAMs with a dominant large cyst causing fetal cardiac failure (hydrops) or resulting in a mass effect severe enough to cause pulmonary hypoplasia or hemodynamic instability from significant mediastinal shift. Placement of a thoracoamniotic shunt diverts cystic fluid from within the CCAM into the amniotic sac and has been shown to reduce CCAM volumes by approximately 70% (12). It can also potentially reverse the hydrops (13).
Under ultrasound visualization, a shunt trocar is percutaneously passed through the maternal abdominal wall and uterus into the amniotic sac near the fetal thorax. The trocar tip is positioned at the superolateral left aspect of a left thoracic macrocyst to encourage superolateral involution of the cyst. The midclavicular line is avoided in order to decrease potential interference with restoration of the normal position
of mediastinal structures (6). The shunt is deployed through the trocar as described for urinary tract obstruction and its position is confirmed by ultrasound.
of mediastinal structures (6). The shunt is deployed through the trocar as described for urinary tract obstruction and its position is confirmed by ultrasound.
This procedure can be complicated by catheter dislodgement, thrombus material occlusion of the catheter, premature rupture of the fetal membranes, preterm labor, fetal hemorrhage, and postnatal rib deformities at placement site (6). Subsequent delivery is managed by routine obstetrical indications and survival rates up to 74% have been reported (14).
Idiopathic Pleural Effusion (IPE)
IPE is another space-occupying lesion of the thorax that produces pathophysiologic effects similar to lung lesions. The resultant mass effect may lead to pulmonary hypoplasia or mediastinal shift with resulting hydrops. Thoracocentesis is performed under ultrasound guidance in order to remove pleural fluid. This fluid is analyzed and lung reexpansion is confirmed following thoracocentesis. Placement of a thoracoamniotic shunt may be indicated if rapid reaccumulation of the pleural effusion occurs. The shunt is placed as described for treatment of a CCAM with the exception that the fetal end of the shunt enters the pleural space and not the fetal lung. The same complications encountered with thoracoamniotic shunt placement for CCAM have been observed with shunt placement for IPE. Overall survival after thoracoamniotic shunt placement is significantly higher compared to that of neonates who did not have a shunt placed (6).
Cardiac Interventions
The final morphologic form of the fetal heart as well as its left and right sidedness is established by approximately 7 weeks of gestational age. Diagnostic ultrasound imaging however, only becomes possible at 12 to 14 weeks of gestation. Congenital heart defects (CHDs) can result from stenotic or atretic valves, leading to hypoplasia of the supporting ventricle that could potentially prevent the ventricle from contributing to postnatal circulation. Cardiac defects appear to result from flow abnormalities through the atrioventricular valves, increased velocities across the semi lunar valves, flow reversal in arterial/venous ducts and aortic isthmus, as well as pulmonary venous flow abnormalities (15). Therefore, the aim of in utero interventions for fetuses with abnormal cardiovascular physiology is to normalize the circulatory imbalance. There is data suggesting that restoration of normal flow promotes growth (16), and that reduction of ventricular pressures minimizes secondary damage by promoting normal development (17). An example of secondary damage is ventricular fibrosis resulting from poor coronary perfusion. Critical aortic stenosis, for example, affords little or no forward coronary artery flow from the aortic root causing coronary perfusion to be dependent on the relatively desaturated arterial duct. Resistance to coronary flow in the fetus is due in part to intraventricular pressure (75%) and myocardial contraction (25%); therefore, the increased end-diastolic ventricular pressure resulting from aortic stenosis would significantly reduce coronary perfusion and promote fibrosis (15).
Percutaneous fetal cardiac interventions that are currently performed include aortic/pulmonary valvuloplasty, balloon atrial septostomy for a restrictive or closed interatrial septum, and fetal pacing for complete heart block (18). Although sonographic guidelines have been developed to aid in selecting appropriate patients for fetal cardiac interventions, the ideal timing of the intervention remains unclear. A number of factors contribute to this uncertainty: First, the stage at which the damage becomes irreversible is unknown; and second, an immature fetus may not be able to tolerate the procedure as the procedure-related risk of death is 10% to 20% and the risk of premature labor is 5% (19).
For most cardiac interventions, once the fetus has received intramuscular analgesia, sedation and muscle relaxation, a long needle with a flexible stylet is percutaneously introduced through the maternal abdomen, uterine wall, and into the fetal chest. Correct alignment of the needle along the axis of the outflow tract is essential, making optimal fetal lie a critical component for success. An over-the-wire coronary balloon is inflated 2 or 3 times across the valve or across the interatrial septum, as tolerated by the fetus (19).
Some fetal complications may arise from a mineralized myocardium that fails to seal at the puncture site leading to hemorrhage. This can potentially lead to cerebral ischemia, cardiac tamponade, or death (20). Of note, the success rate as defined by being able to pass a balloon through the valve has improved to greater than 80% (20); although evidence of improved neonatal outcome is more challenging.
Fetoscopy
Direct endoscopic visualization of the fetus (fetoscopy) was introduced in the 1970s. It was originally used most commonly as a diagnostic tool in order to obtain blood from fetuses with suspected hemoglobinopathies, obtain tissue biopsies, or visualize pathognomonic malformations. The initial real therapeutic application at that time was for intravascular transfusions under direct visualization.
Fetoscopy was not initially widely utilized in the United States due to the invasiveness of the procedure, limited obstetrician involvement, maternal safety concerns, uncertain procedure efficacies, and regulatory difficulties (21). As a result, it eventually became almost obsolete with the widespread introduction of ultrasound in the 1980s.
The real advance in fetoscopy began following funding of the “Eurofoetus” project in 1988, by the European Commission (22). The focus of the project was to evaluate accuracy of routine antenatal ultrasound examination in detecting malformations. The secondary consequence of the project was better collaboration between fetal medicine units and endoscopic equipment manufacturers, resulting in smaller higher quality endoscopes (23). By the 1990s fetoscopic surgery and obstetric endoscopy in combination with ultrasound, began to flourish.
An early clinical application of fetoscopy was for umbilical cord embolization under ultrasound guidance. The first endoscopic cord ligation was for an acardiac–acephalus twin, and was reported in 1993 (24). Unfortunately, a review of experiences with this procedure demonstrated a high rate of preterm premature rupture of membranes (PPROM) (25), and a high occurrence of postoperative amniotic bands (26). This procedure has since been modified to include the use of bipolar forceps, monopolar needles, and transmission of radiofrequency energy using small gauge needles. Other indications for fetoscopy are discussed in subsequent sections.
Placental Sharing
The incidence of twin pregnancies is 1 in 90, of which 30% are monozygotic or identical and 70% are dizygotic or fraternal (27). Seventy-five percent of monozygotic twins are monochorionic or share a placenta. The majority of monochorionic placentas (approximately 96%) have vascular anastomoses that allow blood to flow between both fetuses (27,28,29). This “normal vascular connection” between the twins introduces the possibility of a blood volume imbalance with one twin becoming a donor and “losing blood volume” to the recipient twin who gains blood volume. When a circulatory
imbalance occurs, this placenta sharing becomes pathologic and detrimental to one or both twins in a condition called twin–twin transfusion syndrome (TTTS).
imbalance occurs, this placenta sharing becomes pathologic and detrimental to one or both twins in a condition called twin–twin transfusion syndrome (TTTS).
Twin–Twin Transfusion Syndrome (TTTS)
This condition occurs in approximately 5% to 15% of monochorionic, diamniotic twin gestations with a chronic imbalance in the net flow of blood across the vascular anastomoses. The pathophysiology is usually explained on an angioarchitectural basis. Existing placental anastomoses between twins can be arterio-arterial, veno-venous, or arterio-venous. The first two anastomoses are located superficially on the placental surface and are bidirectional in nature allowing blood flow between the two fetal circulations depending on the relative interfetal vascular gradients. In contrast, arterio-venous communications occur at the capillary level, deep within a shared cotyledon, and are referred to as “deep” anastomoses (Fig. 49-1). This shared cotyledon receives its arterial supply from one twin and its venous drainage (oxygenated blood) drains to the other twin. The arterio-venous anastomoses allow blood flow in one direction only and are identified on the placental surface as unpaired arteries and veins that pierce the chorionic plate at close proximity of each other to supply the underlying shared cotyledon. This unidirectional flow can create an imbalance in interfetal blood flow resulting in twin–twin transfusion. The donor, or pump twin, from whom blood is transfused to the other twin subsequently becomes anemic, hypovolemic, hypotensive, hypoproteinemic, and develops oligohydramnios. Severe intrauterine growth restriction of the donor twin may occur; this together with accompanying oligohydramnios is responsible for this twin also being referred to as the “stuck” twin. The recipient twin however becomes polycythemic, hypervolemic, and is at risk for cardiac failure, hyperbilirubinemia, intravascular thrombosis from blood hyperviscosity, and polyhydramnios from polyuria (30). If this condition is left uncorrected, both twins are at risk for demise.
Twin–twin transfusion (TTTS) is usually diagnosed around 20 to 21 weeks’ gestation although features may be observed at any time during gestation. Diagnosis is either by acute maternal symptoms of polyhydramnios such as abdominal distension, orthopnea, dyspnea, uterine contractions, or by signs observed on recommended serial follow-up ultrasound for monochorionic pregnancies. Ultrasound diagnosis of TTTS includes the presence of polyuria, polyhydramnios, and a distended bladder in the recipient twin with the deepest amniotic vertical pocket measuring at least 8 cm in a pregnancy ≤20 weeks’ gestation or an amniotic pocket of 10 cm after 20 weeks’ gestation. The donor twin is diagnosed by the detection of oliguric oligohydramnios and a maximum deepest vertical pocket of 2 cm (31).
Table 49-2 Quintero Staging of TTTS | ||||||||||||||||||||||||||||||||||||||||||
---|---|---|---|---|---|---|---|---|---|---|---|---|---|---|---|---|---|---|---|---|---|---|---|---|---|---|---|---|---|---|---|---|---|---|---|---|---|---|---|---|---|---|
|
A staging system for TTTS based on ultrasound and Doppler findings was developed by Quintero et al. in 1999 (32). Stage I TTTS is diagnosed by the presence of recipient twin polyhydramnios and donor twin oligohydramnios with a visible bladder in the donor twin. Stage II is also characterized by oligo–polyhydramnios but without a visible donor bladder. Stage III occurs when Doppler blood flow abnormalities exist; these flow abnormalities may include arterial abnormalities in the donor twin (absent end-diastolic velocity in the umbilical artery) or venous abnormalities in the recipient twin such as reverse flow in the ductus venosus or pulsatile umbilical venous flow. The latter may occur with marked tricuspid regurgitation. Stage IV occurs with the development of cardiac failure otherwise referred to as hydrops fetalis in either fetus; and finally Stage V is defined by the demise of a fetus (Table 49-2).
Ultrasound staging does not determine the prognosis of TTTS and some have proposed the use of echocardiographic findings in the recipient twin in conjunction with the staging system by Quintero as prognostic indicators (33,34,35,36) but these have not been found to be of prognostic value either. The treatment of TTTS has evolved over the years and currently includes serial amnioreduction, inter-twin septostomy, selective feticide, and laser photocoagulation of abnormal communicating vessels.
Treatment Options for TTTS
Amnioreduction: Under ultrasound guidance, an 18 G needle is inserted into the amniotic sac of the recipient twin and amniotic fluid is drained until the deepest vertical pocket measures approximately <5 cm. This procedure is repeated serially in order to maintain normal amniotic fluid volume until the fetus is viable, in order to reduce the likelihood of preterm labor from polyhydramnios. Large registries of TTTS pregnancies report a survival rate of 60% to 65% with this technique (37). Management of TTTS with this approach has a risk of serious neurologic sequelae which may occur in up to 20% of babies following delivery.
Inter-twin amniotic septostomy: This involves the insertion of a 20 G spinal needle through the dividing amniotic membrane, under ultrasound guidance, allowing for redistribution of amniotic fluid between the twins. Increased communication between both amniotic fluid sacs may however be complicated by umbilical cord entanglement.
Selective feticide: This is offered as a last resort in the management of TTTS, especially when both fetuses are sick as a result of one of the twins being severely affected. Complete obliteration of all vascular connections to the sick twin becomes mandatory in order to prevent neurologic impairment or demise of the less-affected twin (38).
Medical management: This has included administration of maternal digoxin in order to support cardiac function in the recipient twin. Maternal non-steroidal anti-inflammatory agents have also been used but these have not been reported to improve fetal outcome (30).
Laser photocoagulation of abnormal communicating vessels: This has become a popular indication for fetoscopy. Despite a suggestion for a potential surgical intervention for TTTS by Benirschke and Kim in 1973 (39) and a proposal to use laser energy to coagulate placental anastomoses by DeVore et al. 10 years later (40), it was not until 1990 that De Lia (41) described the technique of laser occlusion of placental vessels in TTTS. This original description involved the insertion of a hysteroscope through a uterine incision made via a mini laparotomy. A simplified percutaneous modification to this technique, performed under local anesthesia, was subsequently reported in 1995 by Ville and colleagues (42). Widespread acceptance for this fetoscopic procedure came from the “Eurotwin2twin” project and publication of a successful clinical randomized trial comparing fetoscopic laser coagulation to amnioreduction for the treatment of TTTS (31). This trial demonstrated a 25% increase in survival and a later delivery time (33.3 weeks vs. 29.0 weeks) with fetoscopic laser coagulation over amnioreduction.
Laser therapy is beneficial for Stage I and II cases of TTTS as less than 10% of cases advance beyond this stage following therapy (43), but it has also proven to be of some benefit in the therapeutic management of Stages III and IV (44). The goal of laser therapy is to selectively photocoagulate all the inter-twin placental vascular anastomoses (arterio-arterial, arterio-venous and veno-venous communications) on the chorionic plate. Under ultrasound guidance, a trocar is inserted into the uterus. A fetoscope is then passed through the trocar and a laser fiber is inserted through the operative channel of the fetoscope. Both neodymium–yttrium aluminum garnet (Nd:YAG) and diode (semiconductor) lasers are used. These lasers convert electrical or chemical energy into light energy leading to the delivery of large amount of energy to a small target area at a distance. There is optimal energy absorbance in the spectrum of hemoglobin; the light from the laser is also absorbed by the hemoglobin content of the red blood cells within the vessels producing volumetric heat. This heat results in coagulation of red blood cells, injury and retraction of the vessel wall, and is diffused into the surrounding placental tissue (Fig. 49-2). Vessels that have been destroyed in this manner demonstrate whitening on macroscopic examination (45).
Complications associated with laser therapy include membrane rupture and premature delivery. When uneven placental sharing is present (10% to 20% of monochorionic placentas), obliteration of all of the connections by laser photocoagulation may remove anastomoses that are compensating for a smaller portion of the placenta and could possibly lead to inadequate placental perfusion of the respective twin (38).
Twin Reverse Arterial Perfusion Sequence (TRAP)
Twin reversed arterial perfusion (TRAP) sequence or “acardiac twinning” is a rare condition (incidence of approximately 1%), which is unique to monochorionic twin gestations (46). In this condition there is an acardiac twin who is kept alive by a structurally normal co-twin referred to as the “pump” twin. Superficial arterial–arterial and venous–venous anastomoses on the placenta permit the pump twin to perfuse the acardiac or recipient twin. The flow is reversed since the recipient twin receives blood via the umbilical artery instead of the umbilical vein. This reversed flow of oxygen-depleted blood from the donor to the recipient twin travels via the uterine artery/arteries to the iliac artery/arteries and the abdominal aorta of the recipient twin. The lower limbs and abdominal organs of the recipient twin are therefore preferentially perfused and become more developed than the upper body which is commonly characterized by absent development of the heart (acardiac) and upper part of the body (anencephalic) in the recipient (47). A hemodynamic disadvantage is placed on the pump twin since this “donor” is providing cardiac output to both itself and the nonviable twin. The pump twin is therefore at increased risk of congestive heart failure from cardiac overload. The rate of perinatal mortality in the pump twin is estimated to be 50% to 75%. Mortality results mainly from polyhydramnios, congestive heart failure, and preterm labor (48). The natural course of monochorionic twin pregnancies with TRAP sequence is uncertain, making optimal management of this condition challenging to determine. The difficulty begins with identifying which pregnancies will benefit from any treatment beyond conservative or expectant management as there is a >50% perinatal mortality risk for the pump twin. Treatment should be mandatory in those cases which show the mildest deterioration in the pump twin on close surveillance scans or in cases of excessive growth of the acardiac twin (49).
Wong and Sepulveda proposed a relatively objective classification system that is based on the abdominal circumference ratio of the acardiac twin to the pump twin, and on signs of compromise in the pump twin who include polyhydramnios, cardiomegaly, and heart failure (Table 49-3). This system is intended to help direct management from expectant follow-up to emergency intervention (50). The classification uses a 50% abdominal circumference (AC) ratio to separate cases into those that may need an intervention following a 2-week follow-up reassessment and those which need prompt or emergent intervention.
Table 49-3 Proposed Management of TRAP Sequence by Classification of Acardiac Anomaly | ||||||||||||||||||||||||
---|---|---|---|---|---|---|---|---|---|---|---|---|---|---|---|---|---|---|---|---|---|---|---|---|
|
Treatment options for the TRAP sequence include expectant management (observation), medical therapies (digoxin, indomethacin), selective delivery, and cord occlusion techniques (embolization, cord ligation, laser coagulation, bipolar and monopolar diathermy). In 2003, Tan and Sepulveda (49) reported on TRAP cases treated with various fetal ablation techniques. They found that following fetal cord ablation, the pump twin overall survival rate was 76%, the gestational age at delivery and median treatment to delivery interval was higher, the technical failure rate was lower, and the rate of premature delivery or rupture of membranes prior to 32 weeks’ gestational age (WGA) was also lower.
Unfortunately incidental demise of the co-twin continues to be a risk in all selective termination procedures. This may occur at the time of the procedure or in the following weeks after the procedure. Other procedure-related complications include preterm labor, preterm delivery, PPROM, and placental or myometrial bleeding (51). Iatrogenic PPROM continues to be the most common complication, occurring in 10% to 30% of cases (26).
Congenital Diaphragmatic Hernia (Cdh)
CDH is characterized by a defect in the diaphragm that permits herniation of abdominal contents into the thoracic cavity. It typically occurs on the left side (84%) although right side and bilateral defects also occur with an incidence of 13% and 2%, respectively (52). The incidence of CDH is estimated at between 1 in 3,000 and 1 in 5,000 live births (53). It is a rare anomaly that occurs in isolation in majority of cases but it may also be associated with other defects. Although CDH is described as a defect in the diaphragm, it is not clear if the primary pathology is pulmonary or diaphragmatic in origin. The clinical consequence of this lesion stems from compression of the developing lung by herniated abdominal contents leading to abnormal lung development. The ipsilateral or affected lung has fewer alveoli, thick alveolar walls, increased interstitial tissue, significantly decreased alveolar air spaces, and decreased gas exchange surface area. The pulmonary
vasculature also develops abnormally with a reduced number of vessels, adventitial thickening, medial hyperplasia, and extension of the muscle layer into the smaller intra-acinar arterioles (54). Survivors of CDH often develop ventilatory insufficiency and persistent pulmonary hypertension, a result of the underlying pulmonary hypoplasia. A dangerous spiral ensues as the abnormal pulmonary vasculature demonstrates increased sensitivity to vasoconstriction, an exaggerated response to hypoxia and hypercarbia and worsening pulmonary hypertension develops and increases the right-to-left shunt. The shunted blood becomes increasingly hypoxic and acidotic which reinitiates the cycle (52).
vasculature also develops abnormally with a reduced number of vessels, adventitial thickening, medial hyperplasia, and extension of the muscle layer into the smaller intra-acinar arterioles (54). Survivors of CDH often develop ventilatory insufficiency and persistent pulmonary hypertension, a result of the underlying pulmonary hypoplasia. A dangerous spiral ensues as the abnormal pulmonary vasculature demonstrates increased sensitivity to vasoconstriction, an exaggerated response to hypoxia and hypercarbia and worsening pulmonary hypertension develops and increases the right-to-left shunt. The shunted blood becomes increasingly hypoxic and acidotic which reinitiates the cycle (52).
Treatment strategies for CDH have evolved over the years (52). Previously, management consisted of aggressive hyperventilation and hyperoxygenation with emergent surgical repair. This transitioned into more gentle ventilation, or even spontaneous ventilation, with permissive hypercapnea to reduce baro- and volu-trauma until a delayed surgical repair was performed. Later, high frequency oscillatory ventilation (HFOV) emerged as a primary therapeutic option either primarily or as a bridge to extra corporeal membrane oxygenation (ECMO). The associated pulmonary hypertension in CDH has been increasingly treated early with inhaled nitric oxide as well as surfactant. These different treatment modalities have impacted the treatment of CDH but have not improved survival in very severe cases resulting in varying survival rates.
A number of features of this condition such as disproportion in ventricular size, degree of mediastinal shift, and stomach position have been considered as possible surrogate markers for indicating the severity of CDH; however, they are poorly validated prognostic indicators (52). As all the problems observed in the neonatal period of a child with CDH seemed to stem from pulmonary hypoplasia, the search began for a way to determine prognosis based on the extent of pulmonary hypoplasia. Metkus and colleagues (55) reported the use of the right lung-to-head circumference ratio (LHR) as a sonographic predictor of survival in fetal diaphragmatic hernia. The LHR is the two-dimensional area of the right lung taken at the level of the four-chamber view of the heart, divided by the head circumference. In a retrospective review of 55 fetuses with left-sided CDH, the LHR was predictive of survival at its extremes: Fetuses with low LHR values of <0.6 did not survive with postnatal therapy. In contrast, those with a value >1.35 had 100% postnatal survival with conventional postnatal therapies including ECMO (56,57). While the accuracy of LHR as described by Metkus and his group has been validated in two prospective studies (58), the LHR has not been widely adopted due to difficulty in accuracy and reproducibility of the measurement (59). It has been observed that there is a rapid growth of normal fetal lung area compared to head circumference particularly between the 12th and 32nd weeks of gestation, therefore Jani et al. (60) proposed referencing the LHR to gestational age by expressing the observed LHR as a ratio to the expected mean LHR for that gestational age. This observed-to-expected LHR ratio (O/E LHR) tends to be more accurate when measured between 32 and 33 weeks. While LHR or O/E LHR is often used to determine the prognosis of CDH, some criticize use of the measurement as it can be operator dependent.
The liver position in CDH (in the chest or in the abdomen) also correlates with survival. However, consideration of the liver as an independent variable that predicts prognosis remains controversial. In general, severely affected fetuses with CDH have an O/E LHR of 25% or less and an intrathoracic liver. Predicted survival in such affected fetuses with a left-sided CDH is approximately 15% (60).
Surgical treatments to reverse the nearly lethal condition of CDH and accompanying severe pulmonary hypoplasia have evolved from in utero anatomical repair to in utero tracheal occlusion. Initial animal studies demonstrated that intrauterine repair of the diaphragmatic defect with reversibility of pulmonary hypoplasia and hypertension was possible but the application to human fetuses was not as promising (61). The observed high maternal morbidity and poor fetal survival caused this method of intrauterine repair to be abandoned. In addition, for fetuses considered to be prime candidates for intrauterine repair due to poor prognosis (those with liver herniation into the thorax), intrauterine attempts to reposition the liver into the abdominal cavity was associated with compression or kinking of the umbilical vein.
Further animal studies demonstrated that obstruction of the trachea during pregnancy allowed for accumulation of tracheal fluid and lung-tissue stretch which in turn stimulated lung growth; thereby decreasing the morbidity and mortality associated with CDH (62). Tracheal occlusion for CDH in humans was subsequently initiated and a number of approaches were utilized including a laparotomy approach with hysterotomy, fetal neck dissection, and tracheal clipping; fetoscopic tracheal dissection and clipping, and more recently, fetoscopic balloon occlusion (52). Initial tracheal balloon occlusion techniques utilized in human fetuses with CDH were however complicated by a high rate of premature labor, and irreversible injury to both the laryngeal nerve and the trachea (58,63). A randomized trial in humans was conducted in 2003 to document the benefit of tracheal occlusion in the management of CDH (64). This study had two arms of CDH patients: Those who underwent in utero fetal tracheal occlusion and those who had postnatal surgical repair. The primary outcome of interest was survival up to 90 days of life and the secondary outcomes were measures of maternal and neonatal morbidity. The study was stopped prematurely because babies in the standard of care arm (postnatal surgery) had an unexpectedly high survival rate of 77% and those in the tracheal occlusion group had a survival rate of 73% at 90 days. It was projected that no statistically significant difference would exist between both arms of the study and it was therefore concluded that in utero tracheal occlusion did not improve postnatal survival or morbidity in neonates. In addition, infants that had in utero tracheal occlusion were noted to have premature delivery at approximately 30 weeks’ gestation, while those allowed to deliver spontaneously, were carried close to term. A criticism of this study was the probability that the prematurity observed in the babies who received intrauterine therapy was due to the fact that a three-port technique was used for the fetoscopic placement of the tracheal clip. Since this early study, extensive research continued in Europe, examining different methods and types of fetoscopic procedures for CDH repair in animals (26,65,66).
Fetoscopic endoluminal tracheal occlusion (FETO) is now an established treatment offered between 26 and 28 weeks’ gestation for human fetuses diagnosed with CDH in Europe (67). It is performed under ultrasound visualization for fetuses with isolated CDH and an LHR <1. The fetus’s position is optimized by external manipulation and following sedation of the fetus, a trocar is inserted through the maternal abdomen and uterine wall. This trocar is exchanged for fetoscopic instruments that include a sheath loaded with an endoscope and the balloon occlusion system. The endoscope is introduced into the fetal trachea and advanced to deliver the inflatable endoluminal balloon to obstruct the trachea (see Fig. 49-3). The balloon is then inflated with fluid.
Following balloon placement, the pregnancy is allowed to continue to near term. Removal of the endoluminal balloon was initially via the EXIT procedure (see EXIT procedure later) under general anesthesia, or by fetal tracheoscopy immediately following vaginal delivery (68). However, it is
now more commonly removed prenatally via ultrasound-guided puncture of the balloon under local anesthesia at approximately 34 weeks prior to delivery (69). Women whose fetuses have undergone the FETO procedure are advised to stay close to the center in which the occlusion was performed in order to facilitate treatment by physicians adept in management of fetal airways should premature labor occur (69).
now more commonly removed prenatally via ultrasound-guided puncture of the balloon under local anesthesia at approximately 34 weeks prior to delivery (69). Women whose fetuses have undergone the FETO procedure are advised to stay close to the center in which the occlusion was performed in order to facilitate treatment by physicians adept in management of fetal airways should premature labor occur (69).
![]() Figure 49-3 Cannula inserted in direction of fetal mouth. The endoscope is then advanced into the fetal pharynx and trachea. Insert: Balloon deposition (images on right). With permission from Deprest J, et al 2006 (70). |
A retrospective study by Deprest and colleagues (57) documented an increase in LHR from 0.7 to 1.7 within 2 weeks of the FETO procedure and a mean gestational age at delivery of 33.5 weeks. The rate of prematurity associated with this procedure has decreased dramatically. In addition, there are few demonstrable clinical side effects of the balloon on the developing trachea except when the balloon is placed early or when complications arise at the time of balloon removal (70). While postnatal tracheomegaly has been observed in some fetuses that have undergone FETO (71), no obvious clinical impact has been documented except for a “barking cough on effort” (72). Fortunately, this widening of the trachea has been observed to become less significant overtime (73).
While FETO serves to stem progress of the lung pathophysiology in utero, definitive surgical repair of the diaphragmatic defect is still necessary following delivery of the baby.
It is estimated that FETO is successful, as measured by placement of the balloon on the first attempt, in 97% of cases. In one study, 97% of cases treated by FETO had subsequent live deliveries although only about half of these survived the subsequent surgery for repair of the diaphragmatic defect and were discharged from the hospital (74). Jani et al. (74) reported PPROM as a significant complication of FETO. It occurs in about 47% of cases, and within 3 weeks of the intervention in about 16%. The risk of PPROM can be predicted by the duration of the procedure as well as the use of general versus regional/local anesthesia; but not by operator experience, gestational age at FETO, side of diaphragmatic defect, placental location, number of FETOs performed, or O/E LHR value (74). Other maternal complications include intra-amniotic hemorrhage and chorioamnionitis. Fetal complications include tracheal laceration and demise although rare.
Fetal Pain and Anesthetic Considerations in the Fetus
Fetal interventions have forced anesthesiologists to shift their paradigm. It was originally believed that only maternal medication was necessary for these procedures. However, the fetus is now accepted as a surgical patient in addition to the pregnant woman. Before discussing anesthesia for the fetus, we must address the issue of the presence or absence of fetal pain and the existing supporting evidence.
Fetal Pain and Analgesia
The concept of fetal analgesia warrants special discussion. Fetal perception of pain and the management thereof, remain an unresolved issue for in utero interventions. Determination of whether or not the fetus feels pain is confounded by a number of issues. First, pain is a subjective experience involving both sensory and emotional components, and it requires a sufficient level of consciousness in order for it to be recognized as “unpleasant” (75). Secondly, the experience of pain is usually associated with a nociceptive stimulus. Phantom-limb pain however, demonstrates that a nociceptive stimulus is not required in order to experience pain; and conversely, reflex withdrawal from noxious stimuli below the level of a spinal cord lesion demonstrates that the feeling of pain does not necessarily have to occur in response to a noxious stimulus (75). Finally, the emotional component of pain implies a psychological construct where conscious perception results from the presence of functional thalamocortical circuitry (76,77).
Establishment of the sensory pathway for the experience of pain begins anatomically at 7 WGA with the formation of peripheral nociceptors that connect to the spinal cord at 8 WGA, and grow C-fibers into the spinal cord by 10 WGA (78). An intact spinal reflex arc responds to non-noxious stimuli at 8 WGA and nociceptive neurons are present in the dorsal horn of the spinal cord by 19 WGA (79). A cerebral cortex, initially isolated from other brain structures, begins to form at 10 WGA (80). A cortical sub plate, which is specific to the fetus, and underlies the cortex from 15 WGA (81), is connected to thalamic afferent fibers around 20 to 22 WGA and subsequently regresses (82). The cerebral cortical plate undergoes a differentiation and maturation process to become the definitive cerebral cortex beginning at 17 WGA and continuing well into postnatal life (83). Penetration of the cerebral cortex by thalamic afferent fibers beginning from 23 to 24 WGA constitutes an intact anatomical pathway for pain (79).
Establishing the sensation of a stimulus and a sufficient level of consciousness, both of which are required in order to recognize the stimulus as “unpleasant,” is more problematic. The very presence of anatomic nociceptive pathways does not establish that the cortex can “perceive” a stimulus and/or “recognize” it as unpleasant. In order for the cortex to
“perceive” a noxious stimulus, both the cortex and the nociceptive pathways must be functional, in addition to being anatomically present. The electroencephalogram (EEG) provides a general assessment of cortical function as it measures summated synaptic potentials from the cortical neurons. A primitive EEG is discernable at 20 WGA, becomes sustained after 22 WGA, and is well characterized from 28 WGA onward (78). Unfortunately, EEG activity alone does not prove that the cortex is functional; since EEG activity may still be present even when functional neural tissue above the brainstem is missing (anencephaly) (84).
“perceive” a noxious stimulus, both the cortex and the nociceptive pathways must be functional, in addition to being anatomically present. The electroencephalogram (EEG) provides a general assessment of cortical function as it measures summated synaptic potentials from the cortical neurons. A primitive EEG is discernable at 20 WGA, becomes sustained after 22 WGA, and is well characterized from 28 WGA onward (78). Unfortunately, EEG activity alone does not prove that the cortex is functional; since EEG activity may still be present even when functional neural tissue above the brainstem is missing (anencephaly) (84).
Somatosensory evoked potentials (SSEPs) demonstrate a cortical response to visceral noxious stimuli, which is transmitted from the nociceptor via the dorsal column tract of the spinal cord through the thalamus to the cortex (85). SSEPs should therefore provide evidence for both cortical and nociceptive pathway function. SSEPs may be elicited beyond 24 WGA and become well developed by 27 WGA (86), suggesting that a functional nociceptive system is present from 24 to 26 WGA.
Determination of a sufficient level of consciousness, required in order to recognize a stimulus as “unpleasant” continues to be unresolved. It has been surmised that consciousness should not be regarded as an all or nothing type of phenomenon, but rather as a continuum, where the level of consciousness may change in a manner similar to a dimmer switch (78). It is believed that consciousness is only possible after an EEG pattern indicates “wakefulness”; an arousal state mediated by the brainstem and thalamus in communication with the cerebral cortex (75). Again, unfortunately, a wakefulness EEG pattern alone does not prove consciousness as this EEG pattern may occur even during a persistent vegetative state (i.e., unconsciousness) (75).
Biophysical markers (e.g., a stress response), which would provide an objective indication of pain, are being investigated. Following a painful stimulus, a stress response (as measured by neuroendocrine or hemodynamic changes) should result and could theoretically be used to infer the perception of pain (87). For example, the plasma concentrations of neuroendocrine stress markers, that is, hormones and neurotransmitters such as cortisol, β-endorphin, and norepinephrine increase in response to a stimulus. It has been shown that following a stimulus the fetus can mount a stress response as measured by increases in both cortisol, β-endorphin (88), and noradrenaline from 18 WGA onward (89). Unfortunately, a neuroendocrine stress response does not prove the presence (or absence) of a painful stimulus as it is mediated by the autonomic nervous system and hypothalamic–pituitary–adrenal axis without conscious processing by the cortex (90). Furthermore, increased fetal plasma concentrations of these markers, for example, noradrenaline, occur following what is considered by some to be non-painful procedures such as umbilical cord transfusions (89,90,91).
Even though an increase in stress response hormones is not absolute proof of a painful experience, it is reasonable to interpret blunting of the stress response as a strong indication that pain was not experienced. It has been demonstrated that opioid analgesia (92) and volatile anesthesia (93) blunt the stress response to surgery in neonates with an improved outcome.
Hemodynamic changes indicating a stress response have been measured using Doppler ultrasound (94) where increased cerebral blood flow (as measured by the pulsatility index in the middle cerebral artery) occurred following venipuncture for fetal blood transfusion via the innervated abdominal wall but not via the non-innervated umbilical cord (95). This redistribution of blood flow toward the brain, at the expense of blood flow to less essential organs (intestine, kidney), referred to as “brain sparing” does not prove the presence of pain as it is also associated with fetal hypoxia (96) and intrauterine growth restriction (97).
Fetal movement in response to touch can be observed from approximately 8 WGA (98) and the experience of pain has been inappropriately attributed to these “withdrawal” movements. A flexion withdrawal response, elicited by cutaneous stimuli, is a spinal reflex that does not involve the cortex; as such, it is a reflex that occurs even in cases of anencephaly (99) and persistent vegetative states (100).
In summary, although the nociceptive pathways are both present and functioning by 24 to 26 WGA, absolute proof that the fetus experiences pain is still lacking. Nevertheless, Lee and colleagues (79) eloquently argue that “… fetal anesthesia and analgesia are still warranted for surgical procedures undertaken to promote fetal health…. evidence of fetal pain is unnecessary to justify fetal anesthesia and analgesia because they serve other purposes unrelated to pain reduction, including (1) inhibition of fetal movement during a procedure, (2)… improve surgical access to the fetus, (3) prevent hormonal stress responses associated with poor surgical outcomes in neonates, ….” Despite the ongoing and persistent debate on whether the fetus feels pain or not, most anesthesiologists will provide analgesia to the fetus during any fetal intervention in which a noxious stimulus is applied directly to the fetus.
Predominant issues for the fetal surgical patient revolve around analgesia, immobility, and hemodynamic stability. Fetal hemodynamic stability will be addressed under maternal considerations.
Anesthetic Considerations for Percutaneous Fetal Intervention in the Fetus
Medications can be administered to the fetus via three general routes: Direct administration, uptake from the amniotic fluid and lastly, via maternal transfer through the placenta. Direct administration can be via the intravenous (umbilical or hepatic vein), intramuscular, or intracardiac routes. The risks of accessing the umbilical vessels include possible compromise of the fetal circulation as a result of vessel dissection, hematoma formation, or thrombus formation. Presumed pain on accessing the hepatic vein can be alleviated with administration of fentanyl (101). The intramuscular route appears to be the preferred route for administration of medication to the fetus unless the umbilical or hepatic vessels are to be accessed for procedural purposes. Intracardiac injection has also been described (102) and may be the preferred route when fetal hemodynamic instability occurs.
Fetal immobility and analgesia is required for intrauterine fetal blood transfusion, cordocentesis, and cardiac interventions since fetal movement may increase the risk of an iatrogenic injury such as inadvertent punctures or needle cuts causing hemorrhage. In addition, access to the target anatomic site, such as is required during deployment of a shunt or balloon, or cardiac interventions may be very challenging in the presence of fetal movement. Fetal immobility and analgesia is therefore achieved with the direct intramuscular administration of nondepolarizing neuromuscular blocking agents and opioids under ultrasound guidance. Pancuronium, the preferred neuromuscular blocking agent due to its vagolytic properties and longer duration of action, can be administered via the intramuscular route (0.2 to 0.3 mg/kg). It may also be administered via the umbilical vein at a dose of 0.1 to 0.25 mg/kg. However, for majority of procedures, which typically last 1 hour, vecuronium may be administered at similar doses via the intramuscular and intraumbilical vein respectively. Opioids, commonly fentanyl (5 to 20 μg/kg) and anticholinergic agents such as atropine and glycopyrrolate can also be administered in combination with nondepolarizing neuromuscular blocking agents. The combination of
these medications in a syringe is handed to the maternal–fetal intervention specialist for administration to the fetus (103).
these medications in a syringe is handed to the maternal–fetal intervention specialist for administration to the fetus (103).
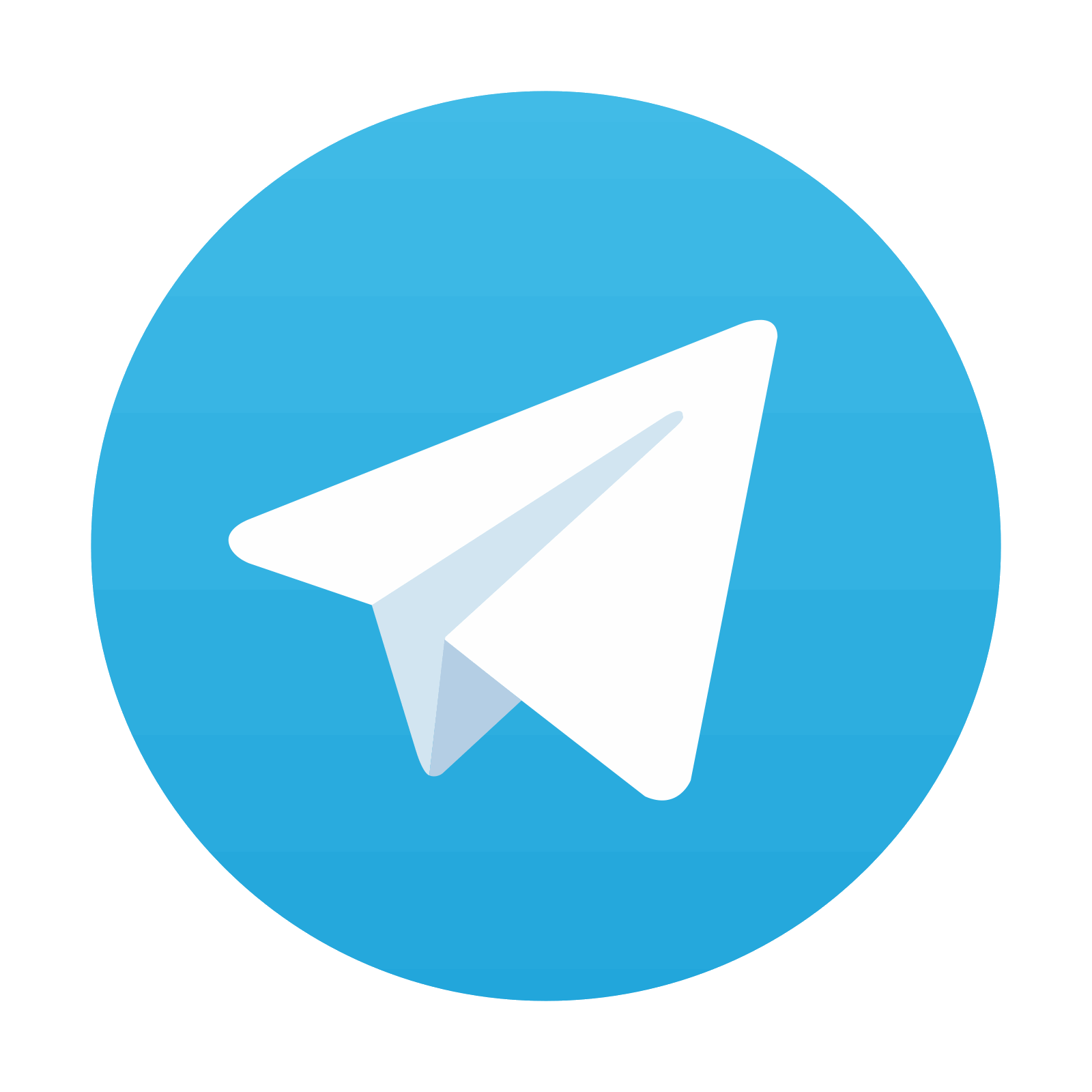
Stay updated, free articles. Join our Telegram channel
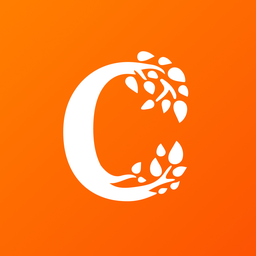
Full access? Get Clinical Tree
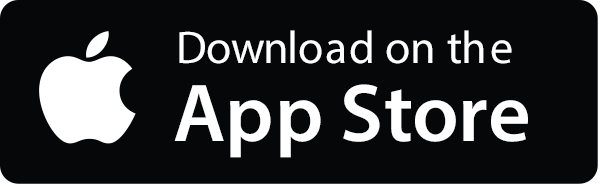
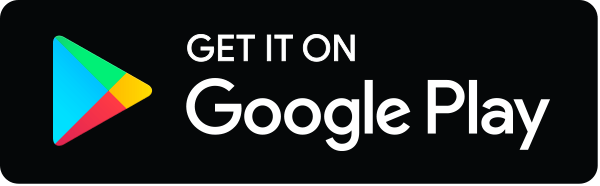