where Hb is hemoglobin concentration (g/dL), SaO2 is arterial Hb–O2 saturation, and PaO2 is arterial oxygen tension.
The second term of Equation (1) represents the dissolved oxygen proportion, which under normal circumstances represents a small fraction of total arterial oxygen content and is therefore often disregarded. However, during HBO this dissolved fraction is substantially increased (Table 41.1). In fact, mixed venous Hb–O2 saturation is 100% under resting conditions while breathing 100% oxygen at 3 ATA. Thus, oxygen delivery can be maintained under these circumstances without hemoglobin, as shown by Boerema in a swine model (10).
PaCO2 is not significantly affected by the increased pressure (8,9), although the venoarterial PCO2 difference is slightly increased, mostly because of a reduction in cardiac output.
Vasoconstriction
Hyperoxia causes peripheral vasoconstriction (8,9). At 3 ATA, systemic vascular resistance (SVR) can increase by up to 40% (9). The mechanisms for this include scavenging of nitric oxide (NO) by superoxide anion (O2−) (11) and increased binding of NO at high PO2 to hemoglobin, forming S-nitrosohemoglobin (9). Vasoconstriction has the positive effect of reducing edema in injured tissues and surgical flaps (discussed later). During HBO, the arterial blood O2 content is sufficiently high that, despite vasoconstriction and reduced blood flow, oxygen delivery is increased (see Table 41.1). Although peripheral vasoconstriction normally occurs during HBO exposure, repetitive intermittent HBO appears to increase the microvascular blood flow of healing wounds (12).
Hemodynamics
Heart rate and cardiac output both decrease by 13% to 35% under hyperbaric conditions (Table 41.2) (8,9), due to activation of baroreceptors by increased blood pressure secondary to systemic vasoconstriction (13). Small changes may occur in systemic and pulmonary artery pressure, with an increase in SVR and a decrease in pulmonary vascular resistance (PVR) (9,14). Despite the reduced cardiac output, oxygen delivery is increased (Fig. 41.1).
TABLE 41.1 Conditions Amenable to Treatment with Hyperbaric Oxygen Therapy |
![]() |
Organ Blood Flow
Animal studies indicate that the decrease in peripheral blood flow is limited primarily to the cerebral and peripheral vascular beds, myocardium, kidney, brain, the ocular globe, and gut. In autonomically blocked conscious dogs at 3 ATA, coronary blood flow is decreased (15). Another dog study at 2 ATA revealed no change in coronary, hepatic, renal, or mesenteric blood flow (16).

FIGURE 41.1 Arterial O2 content and delivery while breathing air at 1 ATA or 100% oxygen at 3 ATA. Measurements are shown in a group of normal volunteers. (From McMahon TJ, Moon RE, Luschinger BP, et al. Nitric oxide in the human respiratory cycle. Nat Med. 2002;8:711–717.)
TABLE 41.2 Blood Gas and Hemodynamic Values in 14 Healthy Adults Breathing Spontaneously (Mean é SD) |
![]() |
Cellular and Tissue Effects
In a myocutaneous flap model during reperfusion following 4 hours of ischemia, Zamboni and colleagues (17) described a delayed decrease in blood flow. This flow reduction appears to be associated with adherence of leukocytes to the endothelium of the small vessels, an effect that is significantly inhibited by HBO. A delayed reduction in cerebral blood flow has also been observed after arterial gas embolism (AGE) in the brain (18), which has similarly been attributed to leukocyte accumulation in the capillaries (19). HBO reduces cerebral infarct volume and myeloperoxidase activity, a marker of neutrophil recruitment (20). HBO also reduces ischemia-induced necrosis in muscle (21,22), brain (23,24), and kidney (25). One mechanism for this effect of HBO appears to be inhibition of leukocyte β2-integrin function (26,27). It has been speculated that part of the beneficial effect of HBO in these settings may be due to prevention of endothelial leukocyte adherence. After focal ischemia, HBO also reduces postischemic blood–brain barrier damage and edema (28) and has an antiapoptotic effect in brain (29) and skin flaps (30).
Antibacterial Effects
The increase in PO2 during HBO can be toxic to anaerobic bacteria, which lack antioxidant defense mechanisms. In addition, HBO has effects on aerobic organisms via neutrophil mechanisms. Killing of aerobic bacteria by leukocytes is related to O2-dependent generation of reactive oxygen species within the lysosomes. In vitro studies have demonstrated that phagocytic killing of Staphylococcus aureus by polymorphonuclear leukocytes becomes less effective as ambient PO2 is decreased. This mechanism appears to be important in vivo when tissue PO2 is low, for example, in osteomyelitis (31). In an animal model of osteomyelitis, the cidal effect of tobramycin against Pseudomonas was increased when tissue PO2 was raised by administration of 100% O2 at increased ambient pressure (32). Published evidence also supports an augmentation of penicillin by HBO in the treatment of soft tissue streptococcal infections (33).
Oxygen Toxicity
Pharmacology
Exposure of an animal to increased partial pressure of oxygen results in higher rates of endogenous production of reactive oxygen species, including superoxide anion (O2−), hydroxyl radical (OH•), hydrogen peroxide (H2O2), and singlet oxygen, which are responsible for tissue oxygen toxicity (34). Tissue O2 toxicity includes the following: lipid peroxidation, sulfhydryl group inactivation, oxidation of pyridine nucleotides, inactivation of Na+-K+ ATPase, and inhibition of DNA and protein synthesis. Toxic effects of these species depend upon both dose and duration of O2 exposure. In the central nervous system (CNS), HBO initially reduces NO availability and causes vasoconstriction. HBO stimulates neuronal NO production and causes the accumulation of peroxynitrite. Prior to onset of a seizure, NO levels and blood flow both increase above control levels (35,36). This, in turn, decreases brain GABA levels, creating an imbalance between glutamatergic and GABAergic synaptic function, which is believed to be partly responsible for CNS O2 toxicity (37).
Clinical Effects
At sufficiently high PO2, any organ can be susceptible to oxygen toxicity. However, within the clinical range of inspired PO2 (1 to 3 ATA), the most susceptible tissues are the lung, brain, retina, lens. and peripheral nerve.
Brain. Oxygen toxicity of the CNS produces a wide variety of manifestations (see Table 41.2) (38). The most common mild symptom is nausea; the most dramatic is generalized nonfocal convulsions. Manifestations of CNS oxygen toxicity are usually self-limited even without pharmacologic treatment and have no long-term effects. The occurrence of a hyperoxic seizure does not imply the development of a convulsive disorder. Factors that increase the risk of CNS oxygen toxicity include hypercapnia and, probably, fever.
CNS O2 toxicity is uncommon when inspired PO2 is less than 3 ATA. While in-water convulsions in divers have been recorded at an inspired PO2 of 1.3 ATA, convulsions during clinical HBO therapy are rare at inspired PO2 partial pressures less than 3 ATA: approximately 0.02% of treatments at an inspired PO2 of 2 ATA and 4% at 3 ATA. At inspired PO2 greater than 3 ATA, the risk of convulsions increases markedly, particularly in patients with sepsis. Although some anecdotal reports suggest that HBO may precipitate seizures in patients who have an underlying predisposition (39), there are no epidemiologic data to confirm this (40). When indicated, HBO should not be withheld on the basis of an underlying seizure disorder.
Both CNS and pulmonary toxicity can be delayed by the use of air breaks (a period of a few minutes where air is administered in lieu of 100% oxygen). Oftentimes, the aura of a hyperoxic convulsion occurs in the form of nausea or facial paresthesias, after which temporary discontinuation of the oxygen (air break) will usually relieve the symptoms and prevent a seizure. Once the symptoms have resolved (usually within a few minutes), the oxygen can be restarted without recurrence. If a seizure occurs, during the tonic/clonic phase the airway may be obstructed. Therefore, it is imperative that chamber pressure not be reduced during this time in order to avoid pulmonary barotrauma and the possibility of AGE. After a convulsion, some practitioners recommend administering prophylactic medication for the duration of HBO.
Prophylactic anticonvulsants such as phenytoin, phenobarbital, or benzodiazepines can reduce the chance of convulsions when utilizing clinical treatment schedules with significant risk of CNS O2 toxicity (e.g., treatment pressure >3 ATA). The authors’ practice is to load septic patients intravenously with phenobarbital as tolerated up to 12 mg/kg prior to HBO treatment at 3 ATA with doses every 8 hours to maintain a serum concentration in the therapeutic anticonvulsant range. When using inspired PO2 less than or equal to 2.8 ATA, the risk of CNS toxicity is sufficiently low that prophylactic anticonvulsant therapy is not required.
Hyperoxic seizures and other CNS manifestations in diabetics can occasionally be caused by HBO-induced reduction in blood glucose (41), caused by increased insulin sensitivity after several consecutive HBO treatments (42). Therefore, the occurrence of CNS O2 toxicity in a patient with diabetes during HBO treatment should prompt the immediate measurement of plasma glucose. When blood PO2 is extremely high, bedside glucose measurement devices, particularly those dependent upon a glucose oxidase reaction, can be inaccurate, producing measurements that significantly underestimate the true value (43); laboratory-based glucose measurement is usually accurate.
Lungs. Pulmonary oxygen toxicity during HBO therapy is also PO2 and time-dependent. Clinical HBO protocols have been empirically developed to minimize the risk of pulmonary O2 toxicity, which almost never occurs during routine daily or twice daily clinical treatments. However, it can occur during extended treatments that are used for treatment of gas embolism or decompression sickness, in which inspired PO2 is as high as 2.8 ATA. The initial manifestation is usually burning substernal chest pain and cough (44), which is most likely due to tracheobronchitis. Most minor pulmonary oxygen toxicity resolves within 12 to 24 hours of air breathing.
Continued exposure to oxygen can produce more severe manifestations such as dyspnea and ARDS. Measurable abnormalities include reduced forced vital capacity (FVC) and carbon monoxide diffusion capacity (DLCO). Complete reversal of FVC decrements as large as 40% of control has been observed after extended O2 exposure at 2 ATA (44). Therefore, in clinical situations requiring aggressive HBO therapy, such as spinal cord decompression sickness or AGE, some degree of pulmonary O2 toxicity is acceptable. Pulmonary oxygen toxicity symptoms may not be evident in patients who are sedated and mechanically ventilated. Moreover, such patients often have pulmonary infiltrates for a variety of reasons and it may be impossible to distinguish the possible additive effects of pulmonary O2 toxicity.
While the maximum safe inspired PO2 during clinical HBO therapy is based mainly upon CNS O2 toxicity limits, the safe exposure duration is determined by pulmonary limits. Prediction formulas have been developed that approximate the average reduction in FVC after continuous oxygen exposure (45,46). However, the usefulness of these algorithms for individual patients is severely limited due to individual variability and comorbid factors that may affect O2 susceptibility, such as prior exposure, intermittent exposure, and endotoxemia. HBO treatment schedules that include periods of air breathing (air breaks) interspersed between O2 periods reduce the rate of onset of both pulmonary and CNS toxic manifestations and can increase the overall dose of oxygen that is tolerated. In the awake patient, the occurrence of burning, retrosternal chest pain is a more useful indicator of incipient pulmonary toxicity.
Supplemental O2 administration at 1 ATA between HBO treatments can accelerate the onset of symptoms of pulmonary O2 toxicity during subsequent HBO. Thus, if O2 is absolutely required between HBO treatments it is prudent to use the lowest concentration possible.
Some antineoplastic agents, such as bleomycin (47,48) and mitomycin C (49), can predispose to fatal pulmonary O2 toxicity, probably due to drug-induced reduction in antioxidant defenses. The risk of pulmonary O2 toxicity due to HBO therapy in patients with previous exposure to either of these agents is unknown, although HBO seems to be safe 3 to 6 months after the agent has been discontinued (50). Even after this point, in some patients, HBO induces mild pulmonary O2 toxicity symptoms such as retrosternal burning chest pain, which can be managed with air breaks.
Eye. Repetitive HBO therapy causes myopia, which is due to a reversible refractive change in the lens (51). A measurable change in visual acuity usually does not occur until after 20 or so treatments. The myopia usually resolves over several weeks, in about the same time period as the onset; however, some residual myopia may remain. Rarely, cataract formation has been attributed to multiple HBO treatments (52), although when this occurs, it is usually after 150 or more hyperbaric sessions (53). Extended exposure to PO2 of 3 ATA can also cause retinal toxicity, manifested by tunnel vision (54,55). However, such exposures are beyond the range used clinically.
Peripheral Nerve. After HBO exposure, some patients experience paresthesias, usually in their fingers and toes, and generally after several HBO exposures but occasionally after a single prolonged treatment. The physical examination is normal and the symptoms resolve within a few hours. This manifestation has no known clinical significance and is not a reason to discontinue HBO therapy.
PHYSICAL EFFECTS OF COMPRESSION AND DECOMPRESSION
Clinically, the complications of HBO therapy that most frequently occur are those related to the body’s gas-containing spaces. Dealing with volume changes in these gas-containing spaces is unique to HBO therapy. For a gas, absolute pressure and volume are inversely related. The increase in pressure during HBO treatment will, therefore, decrease the volume of closed gas-containing spaces within the body, such as the gastrointestinal tract or middle ear (56) and, in the event of gas embolism or decompression sickness, bubbles.
EFFECTS OF GASES OTHER THAN OXYGEN
Nitrogen
Hyperbaric air causes narcosis or pleasant intoxication at pressures greater than about 4 ATA in most individuals, and near unconsciousness at greater than 10 ATA. Since patients breathe oxygen, nitrogen narcosis is only a problem for tenders in multiplace HBO chambers. However, most hyperbaric treatments occur between 2 and 3 ATA, where symptoms of nitrogen narcosis are mild and not noticeable.
Nitrogen and other inert breathing gases such as helium are the causative agents of decompression sickness. During decompression, excess tissue nitrogen can become supersaturated, come out of solution, and form bubbles. This can lead to decompression sickness, with manifestations depending on their location and secondary effects.
Trace Gases
The pharmacologic effects of gases are proportional to their partial pressures. Although a trace gas may only be present in minute quantities, as the chamber pressure rises, so does the partial pressure of a gas. Therefore, gases such as carbon monoxide or carbon dioxide in concentrations that have no pharmacologic or toxic effects at 1 ATA may exert measurable effects in a hyperbaric environment.
HYPERBARIC OXYGEN FOR SPECIFIC DISEASES
Gas Embolism and Decompression Sickness
Gas bubbles in the body can be due to direct gas entry via veins or arteries (arterial or venous gas embolism [VGE]), or due to in situ formation due to gas supersaturation in divers, compressed air workers, or aviators (decompression sickness). Since the two conditions often both occur in the same patient (particularly in divers), and principles of treatment of the two are the same, the syndrome of either or both conditions is commonly referred to as decompression illness (DCI).
Arterial and Venous Gas Embolism
Entry of gas into the circulation can occur via several mechanisms. Gas embolism has recently been reviewed (57,58). In divers breathing compressed gas, AGE can occur if decompression (ascent) occurs while the diver holds his breath or due to gas trapping caused by focal or generalized airways obstruction. AGE due to this mechanism can occur after an ascent to the surface of as little as 1 m. AGE can also occur during diagnostic or therapeutic procedures such as angiography. Retrograde embolization of the cerebral circulation can occur after injection of as little as 5 to 10 mL of fluid containing air bubbles into a radial artery catheter.
VGE can occur due to direct injection or entry via an open vein in which ambient pressure exceeds venous pressure. This can exist during laparoscopic surgical procedures due to the elevated intra-abdominal pressure, or open procedures in which venous pressure in the surgical wound is subatmospheric. The classic scenario for this is an intracranial procedure in the sitting position. However, it has also been described in procedures such as liver resection, cesarean section, and spine surgery. VGE can also occur due to oral hydrogen peroxide (H2O2) ingestion. H2O2 absorbed into the circulation is broken down by catalase into water and oxygen bubbles. VGE can occur if a central venous catheter is opened to air, particularly if the patient is breathing spontaneously. It has also been reported in patients with adult respiratory distress syndrome (ARDS) being ventilated with positive end-expiratory pressure (59). Intravenous (IV) entry is better tolerated than intra-arterial injection because of the pulmonary filter. However, if the rate of entry of gas into the veins is sufficiently high, bubbles can traverse the pulmonary capillary network and become arterial emboli. Large volumes can obstruct the right heart or pulmonary artery and cause cardiac arrest.
Large volumes of arterial gas can cause acute obstruction of large vessels. Small bubbles remain in the circulation only transiently; however, they can precipitate a sustained reduction in local blood flow (18). The mechanism for this appears to be endothelial damage (60) and adherence of leukocytes (19,61,62,63). Endothelial barrier function is also impaired in both the brain and lung, resulting in edema (64,65) and impaired endothelial-dependent vasoactivity (66). Animal models of AGE have revealed significant elevation of ICP and depression of cerebral PO2 (67,68). In a pig model, hyperventilation failed to correct these parameters (69); however, HBO at 2.8 ATA restored both ICP and brain PO2 toward normal.
Clinical manifestations of AGE include acute loss of consciousness, confusion, focal neurologic abnormalities, and cerebral edema. VGE causes acute dyspnea, tachypnea, hypotension, cardiac ischemia or arrest, and pulmonary edema (65). In monitored patients, VGE is often heralded by a decrease in end-tidal PCO2, although sometimes, with small volumes of CO2 embolism such as during laparoscopy, it may be increased. A mill-wheel murmur can be heard in some patients, although this sign is neither sensitive nor specific. Venous gas bubbles in sufficient quantities can cross into the arterial circulation (producing AGE) either through the pulmonary capillary network or via an intracardiac shunt, such as a patent foramen ovale.
Imaging is not useful for diagnosis of either VGE or AGE. Gas bubbles are rarely visible on radiographic images (70). Except in cases where associated conditions such as pneumothorax are suspected, or neurologic conditions such as hemorrhage require exclusion, imaging studies are not necessary and only serve to delay definitive treatment.
Decompression Illness
During diving or exposure to a compressed gas environment such as a hyperbaric chamber, inert gas (usually nitrogen) is taken up by tissues. During decompression, inert gas can become supersaturated and form bubbles in situ in tissues. Certain tissues are more susceptible to in situ bubble formation. Manifestations of DCI can range from mild to severe (Fig. 41.2). The most common manifestations are joint pain and paresthesias. Although mild cases can progress to severe, severe manifestations almost always occur within 12 hours after surfacing.
TREATMENT OF DECOMPRESSION ILLNESS AND ARTERIAL GAS EMBOLISM
Prehospital Treatment
In addition to standard first aid principles, prehospital treatment of DCI consists of administration of a high concentration of oxygen and fluid resuscitation. Oxygen administration reduces bubble size and can sometimes abolish symptoms and signs of DCI. A published study has provided epidemiologic evidence for its efficacy (71). Use of high concentrations of oxygen (preferably 100%) is recommended until definitive treatment is available. Periodic air breaks are appropriate (e.g., 5 minutes every 30 minutes). Administration of oxygen for longer than 12 hours should be based upon the severity of the injury or of the presence of hypoxemia breathing room air.
Both head-down and lateral decubitus positions have been recommended, based on animal studies (72,73). However, the hemodynamic response to VGE is unaffected by body position (74,75) and prolonged head-down position may exacerbate cerebral edema (76). Supine position is, therefore, recommended because patient access and supportive therapies can be more easily administered in this position.
Hospital Treatment
Standard treatment of gas embolism includes airway and ventilatory management, maintaining a high PaO2 and normal PaCO2 (77) (Fig. 41.3) and support of arterial pressure. Like other forms of neurologic injury, it is recommended that when managing neurologic DCI both hyperthermia and hyperglycemia (>140 to 185 mg/dL, 7.8 to 10.3 mM/L) should be avoided or treated (78).
Physical Removal of Gas
Massive VGE has been successfully treated with chest compression (79) and aspiration through catheters in the right atrium (80,81) or pulmonary artery (82).
Recompression. Although symptomatic improvement can be obtained with oxygen at 1 ATA, the definitive treatment of both forms of DCI is HBO (83). The safety and efficacy of HBO for the treatment of divers was initially shown 70 years ago (6). Since then treatment protocols have been empirically developed that have been shown to have a high degree of success with a low probability of oxygen toxicity (84). The most widely used treatment protocols (commonly referred to as tables) were developed by the US Navy and promulgated via the Diving Manual (Fig. 41.4) (85). Both US Navy treatment tables 5 and 6 use 100% oxygen breathing periods (“O2 cycles”) interspersed with air breathing periods (air breaks) at 2.8 and 1.9 ATA in a two-step pattern (see Fig. 41.2). Guidelines are available to administer additional O2 cycles (extensions) at both pressures (85). Most, if not all, cases of DCI can be adequately treated using US Navy treatment tables (83).

FIGURE 41.2 Symptoms of decompression illness in a series of recreational divers. (From Vann RD, Butler FK, Mitchell SJ, et al. Decompression illness. Lancet. 2011;377(9760):153–164.)

FIGURE 41.3 Effect of HBO on ICP and brain PO2 in pigs after air embolism. Top: HBO initially at 2.8 ATA (USN table 6) reduces ICP compared with no treatment, whether it is started 3 minutes or 60 minutes after embolization. Bottom: Brain tissue PbrO2 in the two groups of animals. For the 60-minute group, the closed circles represent PbrO2 in the first 10 minutes after embolization; the open circles represent PbrO2 in the first 10 minutes after the start of HBO. Values in lower panel are mean ± SD. (Adapted from van Hulst RA, Drenthen J, Haitsma JJ, et al. Effects of hyperbaric treatment in cerebral air embolism on intracranial pressure, brain oxygenation and brain glucose metabolism in the pig. Crit Care Med. 2005;33:841–846.)

FIGURE 41.4 A: USN treatment table 5. According to USN guidelines, this table may be used for symptoms involving skin (except for cutis-marmorata), lymphatic system, muscles and joints, with a normal neurologic examination, and when all symptoms have completely resolved within 10 minutes of reaching 2.8 ATA. B: USN treatment table 6. This table may be used for all types of DCI. Extensions (additional oxygen breathing cycles) can be administered at either each treatment pressure (2.8 and 1.9 ATA). (Adapted from Navy Department. US Navy Diving Manual. Revision 6. Vol 5: Diving Medicine and Recompression Chamber Operations. NAVSEA 0910-LP-106–0957. Washington, DC: Naval Sea Systems Command; 2008.)
US Navy tables were designed for use in multiplace chambers, where air breaks can easily be administered by discontinuing O2. Since monoplace chambers were designed to be compressed with 100% O2, shorter alternate treatment tables were designed for their use (Fig. 41.5) (86,87). Although direct comparisons with US Navy tables have never been performed, case series suggest that these tables are efficacious for DCI (87). Monoplace chambers fitted with an air supply and delivery system can be used to administer traditional Navy tables (88).
Adjunctive Measures
In the 19th and early 20th centuries, recompression was the only treatment administered to patients with DCI. While HBO remains the definitive treatment of bubble disease, there is increasing recognition that adjunctive therapies such as correction of hypovolemia may also be important (83,89).
Fluids
Severe decompression sickness is often associated with capillary leak, intravascular volume depletion, and hemoconcentration. Fluid administration to replenish intravascular volume, reverse hemoconcentration, and support blood pressure is a level 1C recommendation (89). IV isotonic fluids without glucose (e.g., lactated Ringer’s, normal saline, or colloids) are recommended for severe DCI. Patients with mild symptoms may be treated with oral hydration fluids. For “chokes” (cardiorespiratory decompression sickness, in which high bubble loads cause pulmonary edema), animal studies suggest that aggressive fluid resuscitation can exacerbate pulmonary edema. Thus, respiratory distress may warrant more judicious fluid administration, particularly if advanced life-support modalities such as endotracheal intubation and mechanical ventilation are not immediately available. For isolated AGE, in which the pathology is limited to cerebral infarction, aggressive fluid administration is also unnecessary.

FIGURE 41.5 Hart–Kindwall monoplace treatment table. This table was designed for use in monoplace chambers without the capability of administering air breaks. Except for the lack of air breaks and limited ability for extension, it is similar to USN table 5, with a shorter time at 2.8 ATA and longer time at 1.9 ATA. (Adapted from Cianci P, Slade JB Jr. Delayed treatment of decompression sickness with short, no-air-break tables: review of 140 cases. Aviat Space Environ Med. 2006;77(10):1003–1008.)
Anticoagulants
Intravascular bubbles can induce platelet accumulation, adherence, and thrombus formation. Although a combination of heparin, PGI2, and indomethacin improved short-term outcome in a canine AGE model (90), the presence of tissue hemorrhage in DCI involving the spinal cord (91,92,93), brain (94,95), and inner ear (96) limits this option.
Patients with leg immobility due to DCI-induced spinal cord injury are at increased risk of deep vein thrombosis (DVT) and pulmonary thromboembolism (PE). Standard prophylactic anticoagulant measures, typically low–molecular-weight heparin (LMWH), are therefore recommended as soon as feasible after the onset of injury (83). Full anticoagulation is appropriate for established DVT or PE. If LMWH is contraindicated, elastic stockings or intermittent pneumatic calf compression is recommended, although their efficacy in preventing DVT or thromboembolism in DCI is unknown. Recommendations have been extrapolated from guidelines for traumatic spinal cord injury; neither their efficacy nor safety in neurologic DCI has been specifically confirmed. Thus, when facilities exist, it is reasonable to perform a screening test for DVT a few days after injury (97).
Lidocaine
The administration of lidocaine in AGE is supported by several animal studies (98). No controlled human studies in accidental AGE have been performed. However, gas emboli are frequently observed in cardiopulmonary bypass. Studies of lidocaine administration in this setting have revealed both improved cognitive outcome (99,100) and no effect (101,102). Human data directly pertinent to DCI are confined to three cases of decompression sickness or AGE, published as case reports, that appeared to benefit from IV lidocaine (103,104). The UHMS does not recommend routine use of lidocaine for DCI; however, if it is used, recommendations have been made for its dosing (97). An appropriate end-point is a serum concentration suitable for an antidysrhythmic effect (2 to 6 mg/L).
NSAIDs
These drugs are commonly used empirically for treatment of bends pain that does not completely resolve with recompression. A randomized controlled trial has been published in which tenoxicam, a nonselective cyclooxygenase inhibitor, was compared with placebo. Tenoxicam or placebo was administered during the first air break of the first hyperbaric treatment and continued daily for 7 days. Using as an end-point the number of hyperbaric treatments required to achieve complete relief of symptoms or a clinical “plateau” of effect, the tenoxicam group required a median of two treatments versus three for the placebo group. The outcome at 6 weeks was not different (105). The UHMS guidelines have assigned NSAIDs a level 2B recommendation (97).
Corticosteroids
Unless given prophylactically, corticosteroids have not been shown to be of benefit in animal models of DCI (106,107,108,109). In a pig model, methylprednisolone treatment did not protect against severe DCI and the treated animals had a greater mortality (110). In the absence of human trials of corticosteroids in DCI and the lack of benefit in animal studies, corticosteroids are not recommended.
Perfluorocarbons
Perfluorocarbons (PFCs) are a family of chemically inert, water-insoluble, synthetic compounds with a high solubility for both inert gases and oxygen, which may eventually become available for human use as blood substitutes. IV injection of PFC emulsions could augment oxygen delivery to ischemic tissues with impaired circulation and facilitate inert gas washout from tissues (111). Indeed, beneficial effects have been observed in animal studies of both decompression sickness and gas embolism (112,113,114,115,116), although some studies have failed to demonstrate an effect (117,118). There may be a benefit from the surfactant properties in the treatment of intravascular gas bubbles (119).
Treatment Summary
Immediate treatment of AGE or DCI includes standard principles of first aid, including administration of oxygen and fluids during transport to a hyperbaric chamber. If the patient is in an extremely remote location from which transport is not feasible and the manifestations are minor, if the patient’s condition does not progress for 24 hours and the neurologic examination is normal, the risk of emergent transport may exceed the risk of conservative treatment (120).
Carbon Monoxide
Carbon monoxide (CO) is an important cause of unintentional poisoning fatalities in the United States each year (121). CO binds to hemoproteins, including hemoglobin and myoglobin, interfering with oxygen transport. It also binds to the mitochondrial cytochrome c oxidase in the electron transport chain (similar to cyanide) impairing oxidative phosphorylation, stopping the cell’s energy production, and resulting in cellular hypoxia, oxidative stress, intravascular platelet–neutrophil activation (122). CO-related oxidative stress can cause chemical alterations in myelin basic protein (123), triggering immune-mediated neurologic deficits.
Sources of CO include internal combustion engine exhaust; appliances such as furnaces, water heaters, and boilers; and charcoal and propane heating and cooking sources. Inhalation of methylene chloride (paint stripper) vapor can cause CO poisoning due to endogenous CO production by the liver.
Symptoms and signs of CO poisoning include headache (or tightness across forehead), weakness, nausea and vomiting, syncope, tachycardia, tachypnea, and encephalopathy. Myocardial ischemia is also common. Exposure to CO in a burning building or metabolic acidosis suggests simultaneous cyanide poisoning.
For survivors of this poisoning, the most debilitating sequelae can be the late neurologic sequelae. These are often cognitive problems such as a decrement in short-term memory (122). Some patients improve clinically and then deteriorate several days after the event.
Oxygen therapy is known to accelerate the elimination of CO (122). The half-life of CO is the longest when breathing air (typically <300 minutes). Half-life decreased to 40 to 70 minutes breathing 100% O2 at 1 ATA (124) and further to 20 minutes with 100% O2 at 2.5 ATA. The reduction in half-life may be important in preventing cell death by allowing mitochondrial ATP production to resume before the cell would have otherwise died. In animal studies, HBO administration after acute CO exposure appears to minimize the lipid peroxidation in the brain which occurs during or after the removal of CO (125) and results in more rapid repletion of brain energy stores (126). A double-blind randomized control trial carried out by Weaver et al. (127) indicates that HBO therapy can prevent occurrence of the late neurologic sequelae of CO poisoning if the patients are treated within 24 hours of the exposure.
All patients should be initially treated with 100% normobaric oxygen. HBO therapy is usually reserved for patients who have more severe poisoning, as determined by high HbCO level (e.g., ≥25%), loss of consciousness or other neurologic manifestation, acidosis, myocardial ischemia, arrhythmias, or other cardiac abnormalities (122,128). A systematic analysis of 163 patients with CO poisoning who did not receive HBO revealed the following two risk factors for sequelae: older age and longer CO exposure (129), although some patients without these risk factors also developed sequelae. The authors concluded that in addition to other indications, regardless of HbCO level or loss of consciousness, anyone older than 36 years with symptoms should receive HBO.
Pregnant women with maternal indications or fetal distress should also be treated. Short periods of HBO therapy are not dangerous to the fetus or mother (122,128,130).
Cyanide
Cyanide leads to hypoxia on a cellular level by rapidly binding to mitochondrial cytochrome oxidase. Inhalation of high concentrations of cyanide (270 ppm) is rapidly fatal in humans (with blood levels reaching 3 μg/mL), whereas ingestion of cyanide is less rapidly fatal. When very low doses of cyanide are absorbed (whole blood levels of 0.5 to 2.53 μg/mL), tachycardia and decreased level of consciousness may occur (131). Measurement of cyanide in the blood is rarely available within the time frame required for therapeutic decision-making, thus the decision to treat must be made presumptively on clinical grounds.
There are very few studies and case reports of the use of HBO therapy in the treatment of cyanide poisoning (132,133,134,135,136). This is likely due partly to the effectiveness of chemical treatments (sodium nitrite and thiosulfate or cyanocobalamin), but also possibly related to that the bonding of cyanide to the mitochondria’s cytochrome c oxidase is not an oxygen-dependent mechanism. Utilizing HBO therapy to increase the amount of circulating dissolved oxygen has been shown to have both prophylactic and antagonistic effects on cyanide poisoning in rabbits (134). Evidence from animal studies suggests that HBO may accelerate washout of cyanide from tissue into blood (137). Human case reports also hint that HBO therapy may be useful when the response to chemical antidotes has been incomplete (135).
Hydrogen Sulfide
Like CO and cyanide, hydrogen sulfide (H2S) reacts with mitochondrial cytochrome c oxidase, impairing electron transport; this is not an oxygen-dependent mechanism. The rationale for using HBO therapy is the same as for cyanide poisoning, in that HBO therapy can increase the dissolved fraction of oxygen. Case reports and a case series suggest that HBO therapy is beneficial for H2S poisoning (138,139,140).
Carbon Tetrachloride
Carbon tetrachloride (CCl4) is a CNS-depressant, hepatotoxin, and nephrotoxin, with renal failure being the most common cause of death from very high-level exposures (141). A case report describes an obtunded patient treated with HBO for presumed CO poisoning. Despite no historical evidence for CO exposure, the patient improved, regained consciousness, and admitted to ingestion of a normally lethal dose of 250 mL of CCl4 (142).
NECROTIZING INFECTIONS
Clostridial Infections
This soil-based anaerobic organism causes a type of rapidly progressive disease known as gas gangrene, which, if left untreated, is almost uniformly fatal. In most cases, it is introduced to the human via accidental trauma. The most common species that cause the disease are Clostridium perfringens (80% to 90%), C. oedematiens, and C. septicum. These organisms release α-toxin, which is a lecithinase related to the form found in snake, bee, and scorpion venoms, causing liquefaction necrosis.
These organisms lack antioxidant defenses and therefore are susceptible to HBO therapy; the first to report this was Brummelkamp (143,144). Around the same time, it was discovered that α-toxin production is inhibited by oxygen at 3 ATA (145). Since then, animal studies (146) and meta-analyses of human case series support the use of HBO (147,148,149).
The typical HBO treatment schedule varies between 2.5 and 3 ATA for 90 minutes with three treatments in the first 24 hours, followed by two treatments per day at 2 to 3 ATA until clinical stability. Aggressive surgical debridement and antibiotic therapy are also essential.
Nonclostridial Bacterial Infections
These are often necrotizing infections, usually polymicrobial, including at least one anaerobic species. These infections often follow local trauma and are enhanced by both local ischemia and reduced host defenses (many patients are diabetic with atherosclerosis). The mainstays of therapy are surgical debridement and antibiotics. Individual case series and meta-analyses support the use of HBO as an adjunct (147,148,149,150,151,152). The HBO treatment schedule is similar to that for clostridial disease.
Mucormycosis
Rhinocerebral mucormycosis is a rare but devastating invasive disease of the head and neck with 30% to 50% or higher mortality when treated, often found in immunocompromised patients such as diabetics in ketoacidosis, or patients receiving antineoplastic agents and/or steroids. It is primarily treated with wide debridement and amphotericin B. Because of the rarity of this disease, randomized trials have not been performed. Several case reports and case series have suggested that HBO therapy may be an effective adjunct (153,154,155,156). Recommended treatment protocol is 2 to 2.5 ATA for 2 hours, twice daily for 40 to 80 treatments.
Severe Anemia
HBO increases dissolved oxygen in the plasma and thus enhances arterial oxygen content. Tissue oxygen delivery can, therefore, be supported acutely even in the absence of hemoglobin. Therefore, HBO at 2 to 3 ATA can be used for temporary support of severely anemic patients if definitive therapy in the form of cross-matched blood is not immediately available (157). Evidence that intermittent repetitive HBO is an effective therapy for patients who refuse blood has no basis in controlled outcome studies (158).
Head Injury
Evidence in animal studies suggests that HBO can prevent secondary injury after head trauma (159). HBO reduces intracranial pressure after head injury in humans (160), possibly due to cerebral vasoconstriction. Small randomized studies have demonstrated improved outcomes with HBO treatment (160,161,162). Beneficial effects of HBO for chronic TBI have not been demonstrated in randomized, double-blind trials (163,164,165,166).
Thermal Injury
In a series of patients with carbon monoxide poisoning due to coal mine explosions and fire, those treated for CO poisoning with HBO who also had burns showed more rapid healing and less infection than others who did not receive HBO (167). Since then, several studies have supported its use (168). It should be noted that thermal burns are often accompanied by acute carbon monoxide poisoning, for which HBO is indicated.
Myocardial Infarction
Increasing the blood O2 content using HBO causes bradycardia and a reduction in cardiac output (8) and myocardial O2 consumption (15). HBO has been shown to improve wall motion abnormalities in patients with resting myocardial ischemia (169). In a rabbit model after 30 minutes of left coronary occlusion, HBO at 2.5 ATA reduced infarct size when administered either during or immediately after occlusion (170). A pilot randomized prospective study revealed lower peak CPK levels and shorter time to pain relief with TPA with a single 2-ATA HBO treatment versus TPA and O2 at 1 ATA delivered via face mask (171). The complete study revealed small, statistically insignificant differences in favor of HBO, but was underpowered to detect differences in mortality (172).
Stroke
A series of 13 patients with stroke treated with HBO at 2 to 3 ATA within 5 hours of onset was published by Heyman (173). At that time no imaging was available to exclude hemorrhage. Nevertheless, of 13 patients treated within 5 hours of symptom onset, 9 improved during HBO treatment and 2 stuporous patients with hemiparesis or hemiplegia improved dramatically immediately upon exposure to HBO and maintained their improvement permanently. The use of HBO in stroke is supported by animal studies demonstrating smaller infarct volume, reduced edema, and attenuation of hemorrhagic transformation (28,174). Human studies have not been encouraging (175), possibly because few, if any patients since Heyman’s study, have been treated within the same short time frame (176). Routine use of HBO in this context will have to await further human outcome studies.
Support of Arterial Oxygenation
HBO has been reported as a method of attempting to support arterial blood oxygenation in RDS of the newborn, with disastrous results because of pulmonary oxygen toxicity (177). HBO is occasionally used for short periods to support oxygenation during therapeutic lung lavage (178–181).
Sedation and General Anesthesia During Hyperbaric Treatment
Anesthetic agents may be required for surgery while in a saturation diving system (e.g., offshore), for therapeutic lung lavage or for sedation during mechanical ventilation. Inhaled agents can be used with conventional anesthetic vaporizers, which deliver a constant partial pressure of agent irrespective of chamber pressure. Nitrous oxide can be used as a sole agent at increased pressure, although it induces several disagreeable side effects, including tachypnea, tachycardia, hypertension, diaphoresis, muscle rigidity, catatonic jerking of the extremities, eye opening, and opisthotonus. It is also associated with severe nausea and vomiting after recovery (182). Nitrous oxide must be avoided entirely in helium atmospheres because its administration induces intravascular bubble formation due to isobaric counterdiffusion through the skin (183). Nitrous oxide should also be avoided even at 1 ATA in patients who have recently scuba-dived or experienced DCI. In such patients tissue bubbles may be present, which could enlarge due to NO diffusion and cause symptoms (184).
Inside hyperbaric chambers, IV agents such as propofol, ketamine, midazolam, and narcotics are preferred because their use avoids atmospheric pollution. Pressure-induced reversal of anesthesia is not significant up to 10 ATA and if it occurs at higher pressures, it can be offset by appropriate titration.
HYPERBARIC CHAMBER OPERATION
Types of Hyperbaric Chambers
Monoplace
As implied by the name, these chambers have space for only one average-sized adult. Generally speaking, modern chambers of this type are cylindrical in shape and made of a large (approximately 0.6 to 1 m internal diameter and 2.1 to 2.3 m long) clear acrylic tube with a cap on one end and entry/exit hatch on the other. Patients slide into the chamber through the hatch to rest supine while they receive HBO therapy (Fig. 41.6).

FIGURE 41.6 Monoplace chamber. This type of chamber has room for one patient or a tender with a small child. Chamber atmosphere is 100% O2. The chamber is constructed of transparent Plexiglas to allow observation. Through-hull penetrators in the door on the left can be seen and allow monitoring, intravenous fluid administration and control of a ventilator inside the chamber. (Courtesy of Dr. Lindell Weaver.)
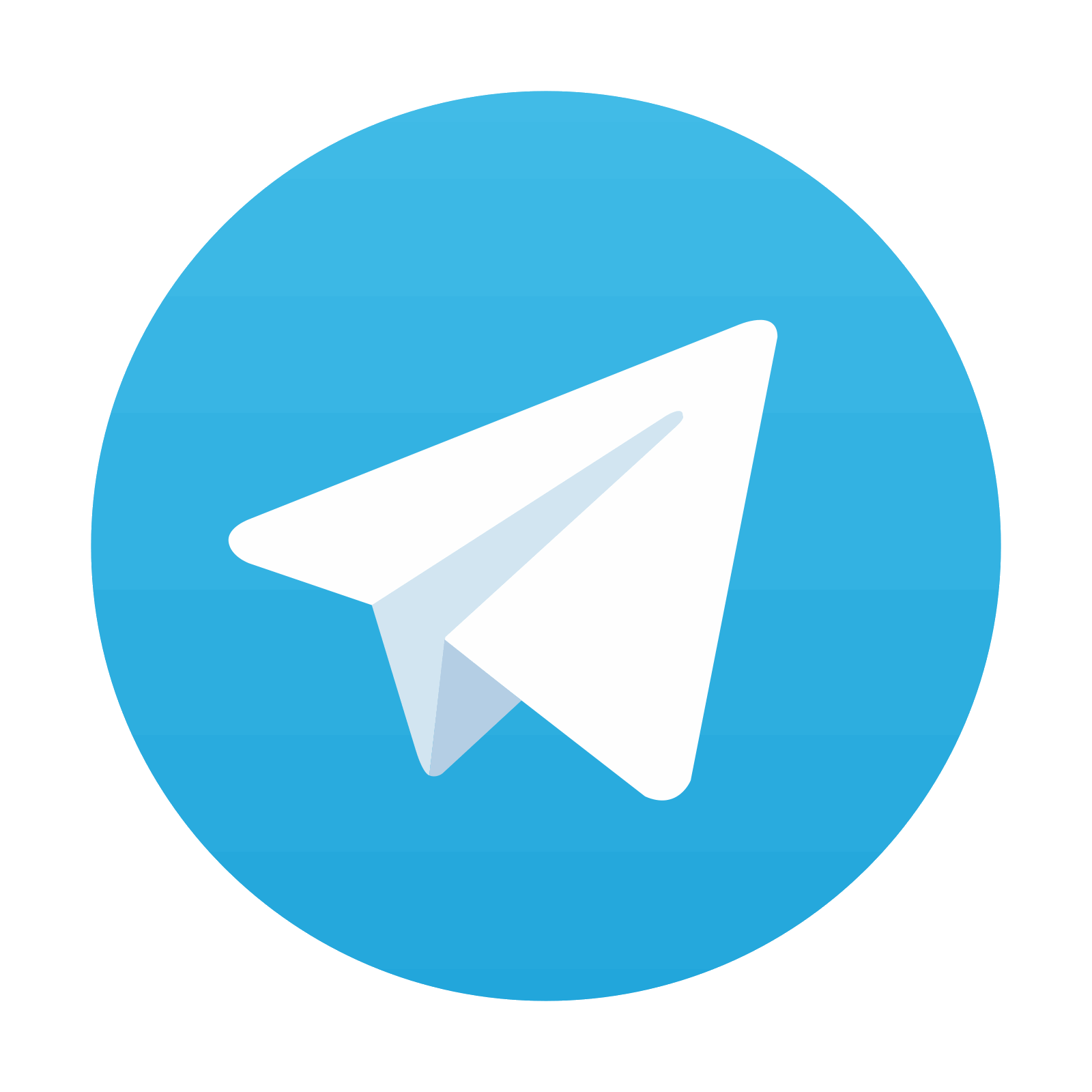
Stay updated, free articles. Join our Telegram channel
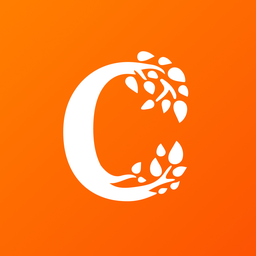
Full access? Get Clinical Tree
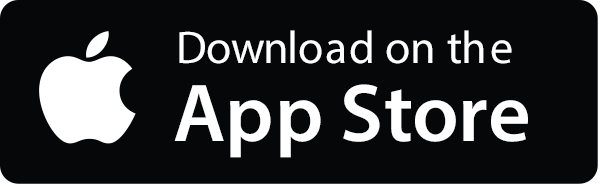
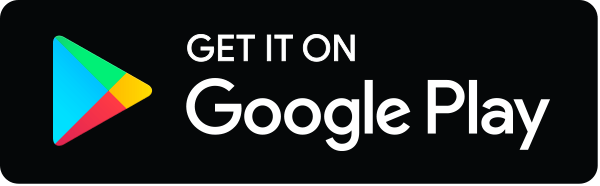