Globally increased metabolism fuels critical illness. The body provides and consumes basic substrates taken from its own structures to run at an accelerated metabolic rate, a rate that cannot be indefinitely sustained. Clinicians in the critical care setting are familiar with the long-term consequences of catabolic processes in patients whose illness is not alleviated; outcomes are poor and mortality is high. This archetype contrasts with a stress response that transitions to a later period of recovery and anabolism with recovery. Patterns vary, but all critically ill patients experience increased metabolism in a neurologic, hormonal, and immunologic milieu that reprioritizes many functions of the healing process. This process is adaptive and, in prolonged and uncontrolled situations, pathogenic.
Cuthbertson was among the first to describe and explain the stress response, a pattern of metabolic changes in injured patients. In his framework, the physiology of response was viewed as adaptive. Metabolic changes from “normal” were thought to be necessary to heal serious injury. As part of this process, patients might become ill enough to require aggressive therapy. Indeed, in patients with underlying comorbidities, intervention to correct or reverse the stress response might be helpful. Unfortunately, although the metabolic changes induced by “stress” have been described, their meaning remains subject to interpretation, and intervention must be undertaken with trepidation. Our understanding of most aspects of the metabolic response to injury is, by and large, characterized by a dearth of clear evidence, a glut of theory, and an absence of consensus. This chapter considers the predictable pattern in response to injury, the interventions that alter this pattern, and the diagnostic utility of comparing a patient’s clinical data to a generalized stress response pattern.
Pathophysiology and Mechanism of Action
Cells metabolize glucose, lactate, amino acids, fatty acids, ketones, and their derivatives. They assemble these components into larger carbohydrates (glycogen), proteins, and triglycerides for energy storage and cellular function. Anabolic processes assemble small molecules into larger ones and consume energy. Catabolic processes deconstruct these larger molecules and release energy. Thus, large molecules can be viewed as sites of stored energy.
Catabolism, the hypermetabolic recovery period of “flow” that follows the “ebb” of shock in Cuthbertson’s original description, is the trademark of critical illness. These two phases are followed by a third: an anabolic recovery phase that commences after resolution of the stress response and persists for weeks to months ( Fig. 60-1 ). Changes affect the entire body, alter activity in each organ system, and are reflected in secondary dysfunction in these systems. Available evidence supports the theory that this adaptive response enables tissue healing.

Resting energy expenditure increases in critical illness. Glucose and fatty acids are consumed at accelerated rates. Serum levels of both exceed the normal range. Proteins are catabolized to amino acids, which in turn are converted by the liver to glucose. Patients develop hyperglycemia. Levels of lactate increase because of a metabolic shift and do not necessarily reflect tissue hypoperfusion, as is the case in acute shock. The catabolism of stress is not the same as that of starvation. In the former tissue, protein is consumed preferentially rather than spared. The liver produces acute phase reactants, such as C-reactive protein, immunoglobulins, fibrinogen, and haptoglobin, often at the expense of other proteins such as prealbumin, albumin, and transferrin. Muscle tissue provides most of the amino acids for fuel and protein synthesis. Ketosis is rare because hyperglycemia stimulates insulin release and insulin suppresses ketogenesis. Provision of exogenous substrate cannot completely attenuate the loss of body proteins. The intestines continue to absorb glutamine, but conversion to citrulline drops, suggesting nutritional reprioritization. Serum amino acid profiles change in septic patients with their illness trajectory. These findings underscore the fact that nutrient use is altered during critical illness and that metabolic priorities are changed. Critically ill patients will not respond to endocrine, nutritional, or metabolic therapies in the same way that unstressed patients do.
Some end-organ cells lose part of their ability to oxidize fuels in the mitochondria. For these cells, metabolism and oxygen use decrease, leading to a metabolic “shunt” and organ dysfunction. This bioenergetic failure correlates with illness severity. It may be adaptive, and recovery is sometimes possible, but the resulting organ dysfunctions frequently require supportive interventions.
Endocrine and neurologic axes drive part of the change in metabolism. The anterior pituitary releases large amounts of growth hormone, thyroid-stimulating hormone, luteinizing hormone, and prolactin, but there is peripheral resistance to normal metabolic effects. Metabolism and cardiovascular function change as a consequence of elevated levels of catecholamines and vasopressin. Although insulin, glucagon, and cortisol levels are increased, their anabolic effects are attenuated.
Changes in organ systems function characterize the acute phase of critical illness. Recognition of these findings sometimes heralds a new diagnosis, such as sepsis, and should provoke clinicians to increase monitoring and perhaps begin empiric therapy.
Neurologic. Brain tissue uses a wide variety of metabolic fuel. During stress, glucose, amino acid, and lactate metabolism increases. Encephalopathy frequently develops, possibly related to the presence of elevated levels of aromatic amino acids and their metabolites. Global cerebral function declines, manifested as alterations ranging from delirium to overt coma.
Cardiovascular. Stress accelerates oxygen consumption in the periphery. To compensate, cardiac output increases and peripheral vascular tone decreases, augmenting blood flow to peripheral tissues, possibly at the expense of flow to other vascular beds. That oxygen consumption is highest in leukocyte-dense tissues suggests that heightened oxygen delivery is destined for cells that repair tissue and control infection. Capillary beds leak because of alterations in glycocalyx function. The balance between fluid extravasation and reabsorption favors the formation of edema because plasma proteins accumulate outside vessel walls, pulling fluid and electrolytes with them.
The result of these changes is a hyperdynamic circulation and accumulation of edema. In some patients, myocardial injury may ensue. Damage may lead to a failure to supplement oxygen delivery, which is associated with a high mortality in critical illness lung injury. Although adaptive, there is little associated benefit from attempts to enhance this response by supplementing oxygen delivery.
Fluids, Electrolytes, and Nutrition. Tissue edema and intravascular resuscitation increase body water, and patients characteristically gain weight. The distribution of water during the stress response was explored by Moore and colleagues. The extracellular and vascular compartments expand, but intracellular water is lost. This effect suggests that water shifts from the intracellular space to the extracellular space and back again as edema resolves, which has implications for electrolyte balance.
Insensible water loss and diuretic use produce hypernatremia. Sometimes renal water retention dominates, causing hyponatremia. As the stress response abates, water shifts back into the intracellular space, stimulating a concomitant inward flux of potassium, magnesium, phosphate, and proteins from the plasma. Thus hypokalemia, hypomagnesemia, and hyperphosphatemia are common. Hypermetabolism or the refeeding syndrome also may cause hypophosphatemia.
Whole-body glucose delivery increases as a consequence of decreased peripheral uptake and increased production. Hepatic gluconeogenesis converts amino acids and glycerol to glucose, even during hyperglycemia. Amino acids, freed from peripheral protein stores, feed this process. It is likely that the driving force for increased glucose production and decreased utilization is leukocyte demand in injured areas. Hyperglycemia and edema formation deliver glucose to relatively avascular injured areas. Lipid metabolism increases in the stress response, but not as much as triglyceride hydrolysis and re-esterification, resulting in elevated serum triglycerides. Many fat stores undergo mobilization and subsequent recomposition.
Pulmonary. Pulmonary insufficiency accompanies the stress response. Enhanced oxygen consumption and carbon dioxide production put greater demand on the pulmonary system. Tachypnea and type I (oxygenation) and type II (ventilation) failure occur. Perivascular flux forces fluids and proteins into alveoli. Inflammatory infiltration exacerbates extravasation in certain patients. Altered immune function and risk for aspiration foment pulmonary infection. Such changes culminate in pulmonary dysfunction, including the adult respiratory distress syndrome.
Gastrointestinal. Intestinal villi atrophy and the gut swells. Ileus heralds worsening stress. These changes confound attempts to provide enteric nutrition and increase the risk of bowel obstruction. Hepatic metabolic changes include impaired excretion of bilirubin and other metabolites.
Renal. Peripheral vasodilatation steals blood flow from the kidneys. Reduced perfusion and circulating mediators produce a syndrome of oliguria. Metabolically active tubular cells suspend function and become quiescent until the stress has long resolved. The extreme example of this condition is acute kidney injury. With recovery, renal function usually returns.
Immunologic. Cell-mediated immunity is classically suppressed during inflammation. Susceptibility to infection increases as systemic inflammatory signals are elevated.
Endocrine. Cortisol, catecholamines, and glucagon drive part of stress hyperglycemia. Relative cortisol deficiency worsens vasodilatory shock and stalls recovery. The peripheral response to insulin changes with immunologic signaling in peripheral muscle and fat. Changes in endocrine signaling include the euthyroid sick syndrome, disorders of sleep cycles, and altered immunologic function. Eventually, pituitary hypersecretion and altered peripheral sensitivity may give way to exhaustion.
If critical illness continues unabated, then metabolic signaling changes. Adiponectin levels decrease with acute critical illness, but they then normalize as illness continues, suggesting changing immune and metabolic signaling. In this setting, catabolism continues and may be increased. Anterior pituitary hormone levels decline in a functional state of neuroendocrine exhaustion.
Ongoing stress leads to a state of persistent critical illness. In this state, neuroendocrine exhaustion is compounded by widespread bioenergetics/mitochondrial failure, organ dysfunction, kwashiorkor-like protein malnutrition, and a stymied immune response. Regular biologic oscillators lose their signaling, adiponectin levels increase again, and tissue macrophages adopt an “anti-inflammatory” phenotype. These patients are susceptible to infection and are dependent on life-sustaining organ support therapies.
Recovery from any of these phases entails source control followed by prolonged anabolism. Tissue protein stores slowly recover. It takes time to replete proteins, endocrine axes, and immune responses to their normal function. Myopathies, neuropathies, and wound healing are the most visible manifestations of the slow recovery.
Available Data
Several clinical trials suggest ways in which the physiology of critical illness is mediated and how attempts to interfere with it might help or harm. Herndon et al. studied the use of beta-blockade in burned children to reduce the loss of muscle mass. They found that large doses of propranolol (average 6.3 mg/kg/day) produced a 6% absolute difference in lean body mass after 2 weeks of hospitalization. This trial examined the role of catecholamines in stress hypermetabolism and showed that blockade of rampant protein catabolism might improve outcomes in certain settings.
Adrenal hormone replacement may treat septic shock and other forms of acute critical illness, but the metabolic and immunologic consequences of this therapy are difficult to disentangle. Large doses of cortisol worsened mortality. One study reported increased survival with selective administration of lower doses of cortisol in patients in whom a cosyntropin stimulation test signaled impaired response. Other investigators have found no survival benefit. The value of diagnostic tests for adrenal insufficiency has been questioned because protein binding is so variable among critically ill patients. Current evidence shows little benefit from therapeutic administration of steroids to patients during the stress response. This volume contains a comprehensive discussion of the topic elsewhere.
The amount of nutrition needed during critical illness is an ongoing source of debate. Nutritional support modestly prevents excessive protein loss, hyperglycemia, or hyperlipidemia and may improve organ and immune function. The available literature is highly prone to bias. A few findings are consistent. Very high levels of nutrients are associated with worse outcomes, “underfeeding” may be beneficial, and parenteral nutrients do not have the same effects as enteric nutrition. Nutritional topics are treated elsewhere in this text.
Attempts to alter stress metabolism can have adverse consequences. Although administration of anabolic hormones might attenuate loss of lean muscle mass and improve outcomes in critical illness, studies of androgen supplementation show mixed results. In one study, growth hormone supplementation increased mortality. In a surgical population, aggressive insulin therapy improved survival and reduced organ dysfunction when serum glucose was driven to nonstress levels. A subsequent study of patients in a medical intensive care unit by the same investigators and studies by others failed to replicate these results. The original study was criticized for its large proportion of cardiac surgery patients, the restriction of benefit to patients who stayed in the ICU longer, and the aggressive nutrition given to the patients. Given the changes and variability in stress metabolism during critical illness, it is conceivable that the goals of insulin therapy should vary with a patient’s position on the stress curve such that catabolism is not overly suppressed early and anabolism is supported late. This concept remains unstudied.
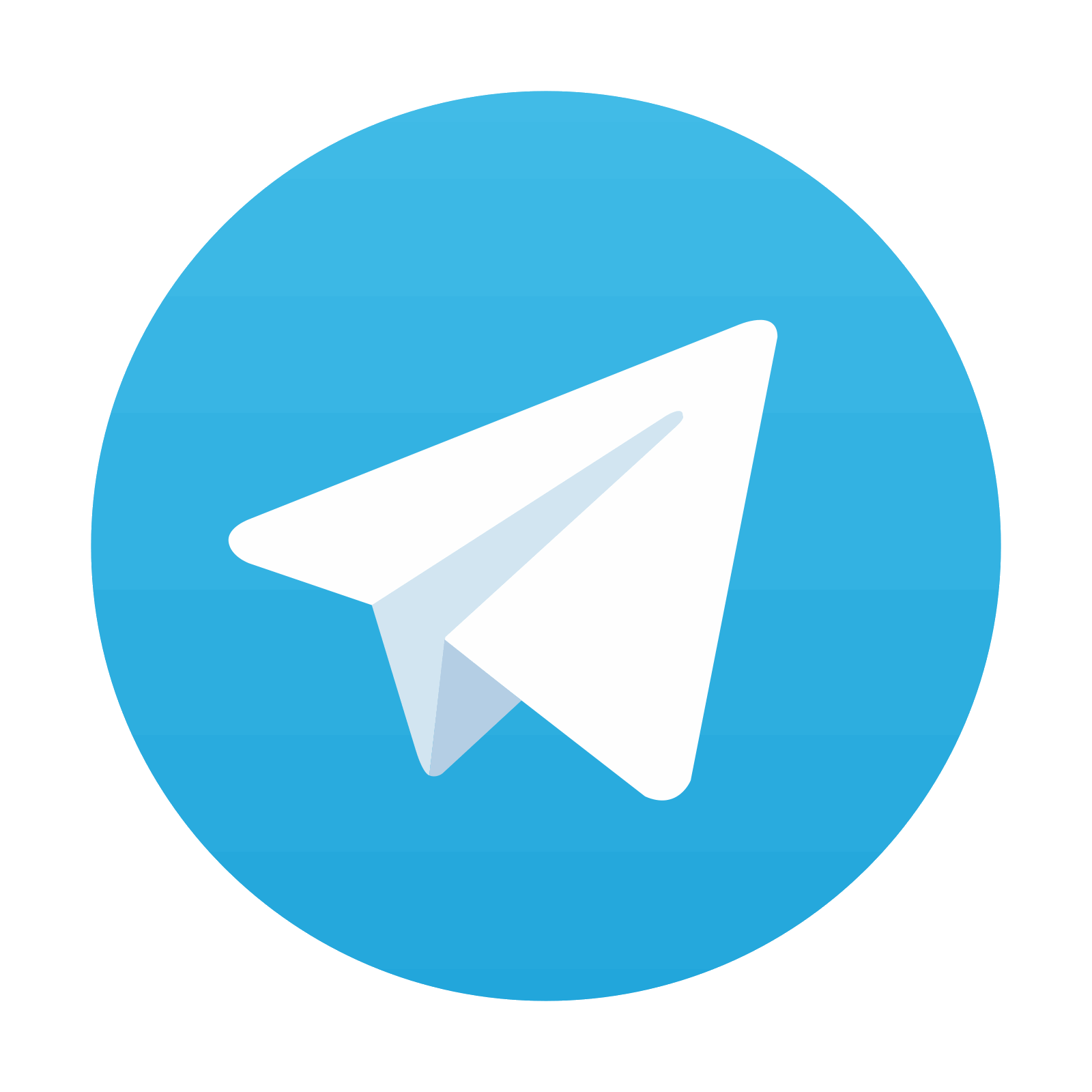
Stay updated, free articles. Join our Telegram channel
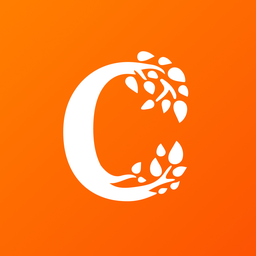
Full access? Get Clinical Tree
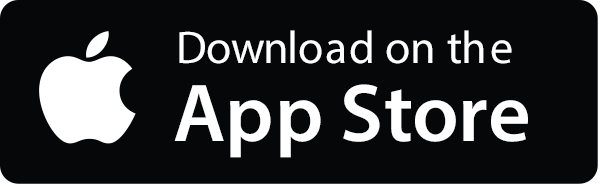
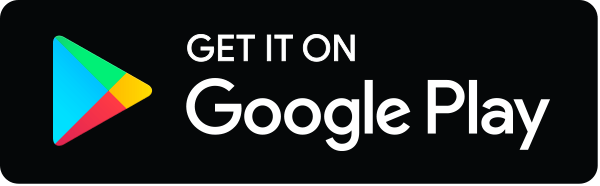