Chapter 1 High-Altitude Medicine and Physiology
The Population
More than 40 million tourists visit recreation areas above 2400 m (7874 feet) in the American West each year. Hundreds of thousands visit central and south Asia, Africa, and South America, many traveling to altitudes above 4000 m (13,123 feet). In addition, millions live in large cities above 3000 m (9843 feet) in South America and Asia. The population in the Rocky Mountains of North America has doubled in the past decade; 700,000 live above 2500 m (8202 feet) in Colorado alone. Increasingly, physicians and other health care providers are confronted with questions of prevention and treatment of high-altitude medical problems (Box 1-1), as well as the effects of altitude on preexisting medical conditions. Despite advances in high-altitude medicine, significant morbidity and mortality persist (Table 1-1). Clearly, better education of the population at risk and those advising them is essential. Altitude illness should be considered a public health problem. In this chapter, we emphasize clinical issues likely to be encountered in lowlanders visiting high-altitude locations by reviewing basic physiology of ascent and the pathophysiology, recognition, and management of medical problems for both visitors and residents of high altitudes (see Box 1-1).
BOX 1-1 Medical Problems of High Altitude
Definitions
High Altitude (1500 to 3500 m [4921 to 11,483 Feet])
The onset of physiologic effects of diminished partial pressure of inspired oxygen (PIO2) includes decreased exercise performance and increased ventilation (lower PaCO2) (Box 1-2). Minor impairment exists in arterial oxygen transport (arterial oxygen saturation [SaO2] at least 90%), but PaO2 is significantly diminished. Because of the large number of people who ascend rapidly to 2500 to 3500 m (8202 to 11,483 feet), high-altitude illness is common in this range (Table 1-2; see Table 1-1).
BOX 1-2 Glossary of Physiologic Terms
PB | Barometric pressure* |
PO2 | Partial pressure of oxygen |
PIO2 | Inspired PO2 (0.21 × [PB − 47 mm Hg] |
(47 mm Hg = vapor pressure of H2O at 37° C [98.6° F]) | |
PAO2 | PO2 in alveolus |
PACO2 | PCO2 in alveolus |
PaO2 | PO2 in arterial blood |
PaCO2 | PCO2 in arterial blood |
SaO2 | Arterial oxygen saturation (HbO2 ÷ total Hb × 100) |
RQ | Respiratory quotient (CO2 produced ÷ O2 consumed) |
Alveolar gas equation | PAO2 = PIO2 − (PACO2/RQ) |
Very High Altitude (3500 to 5500 m [11,483 to 18,045 Feet])
Maximum arterial oxygen saturation falls below 90% as PaO2 falls below 50 mm Hg (Figure 1-1; see Table 1-2). Extreme hypoxemia may occur during exercise, sleep, and high-altitude pulmonary edema or other acute lung conditions. Severe altitude illness occurs most commonly in this range.
The Environment of High Altitude
Barometric pressure falls with increasing altitude in a logarithmic fashion (Table 1-3). Therefore the partial pressure of oxygen (21% of barometric pressure) also decreases, resulting in the primary insult of high altitude: hypoxia. At approximately 5800 m (19,029 feet), barometric pressure is one-half that at sea level, and on the summit of Mt Everest (8848 m [29,029 feet]) the inspired pressure of oxygen is approximately 28% that at sea level (see Figure 1-1 and Table 1-3).
TABLE 1-3 Altitude Conversion: Barometric Pressure, Estimated Partial Pressure of Inspired Oxygen, and the Equivalent Oxygen Fraction at Sea Level*

The relationship of barometric pressure to altitude changes with distance from the equator. Thus, polar regions afford greater hypoxia at high altitude in addition to extreme cold. West565 has calculated that barometric pressure on the summit of Mt Everest (27 degrees N latitude) would be about 222 mm Hg instead of 253 mm Hg if Mt Everest were located at the latitude of Mt McKinley (62 degrees N). Such a difference, he claims, would be sufficient to render impossible an ascent without supplemental oxygen.
In addition to the role of latitude, fluctuations related to season, weather, and temperature affect the pressure-altitude relationship. Pressure is lower in winter than in summer. A low-pressure trough can reduce pressure 10 mm Hg in one night on Mt McKinley, making climbers awaken “physiologically higher” by 200 m (656 feet). The degree of hypoxia is thus directly related to barometric pressure, not solely to geographic altitude.565
Temperature decreases with altitude (average of 6.5° C [11.7° F] per 1000 m [3281 feet]), and the effects of cold and hypoxia are generally additive in provoking both cold injuries and high-altitude pulmonary edema.419,559 Ultraviolet light penetration increases approximately 4% per 300-m (984-foot) gain in altitude, increasing the risks for sunburn, skin cancer, and snowblindness. Reflection of sunlight in glacial cirques and on flat glaciers can cause intense radiation of heat in the absence of wind. We have observed temperatures of 40° to 42° C (104° to 107.6° F) in tents on both Mt Everest and Mt McKinley. Heat problems, primarily heat exhaustion, are often unrecognized in this usually cold environment. Physiologists have not yet examined the consequences of heat stress or rapid, extreme changes in environmental temperature combined with the hypoxia of high altitude.
Acclimatization to High Altitude
Recently, a revolution has taken place in our understanding of the molecular mechanisms of human responses to hypoxia. At the center of this rapidly changing field is hypoxia-inducible factor (HIF), a transcription factor that modulates the expression of hundreds of genes, including those involved in apoptosis, angiogenesis, metabolism, cell proliferation, and permeability processes.90,423,484–487,503,504 In chronic hypoxia, HIF activation by hypoxia has the positive effect of elevating oxygen delivery by boosting hemoglobin mass. But HIF also plays a role in carotid body sensitivity to hypoxia, which in turn largely determines the ventilatory response to hypoxia. As a master regulator of the hypoxia response in humans, HIF has some beneficial and harmful effects at different stages in the time course of human exposure to hypoxia, and in different cells in the body. See Figure 1-2 for an overview of some of the hundreds of processes in which the response to hypoxia is modulated by HIF.
Individuals vary in their ability to acclimatize, no doubt reflecting certain genetic polymorphisms, including HIF. Some adjust quickly, without discomfort, whereas acute mountain sickness (AMS) develops in others, who go on to recover. A small percentage fails to acclimatize even with gradual exposure over weeks. The tendency to acclimatize well or to become ill is consistent on repeated exposure if rate of ascent and altitude gained are similar, supporting the notion of important genetic factors. Successful initial acclimatization protects against altitude illness and improves sleep. Longer-term acclimatization (weeks) primarily improves aerobic exercise ability. These adjustments disappear at a similar rate on descent to low altitude. A few days at low altitude may be sufficient to render a person susceptible to altitude illness, especially high-altitude pulmonary edema (HAPE), on reascent. The improved ability to do physical work at high altitude, however, persists for weeks.317 Persons who live at high altitude during growth and development appear to realize the maximum benefit of acclimatization changes; for example, their exercise performance matches that of persons at sea level.365
Ventilation
By reducing alveolar carbon dioxide, increased ventilation raises alveolar oxygen, improving oxygen delivery (Figure 1-3; see Figure 1-1). This response starts as low as 1500 m (4921 feet) (PIO2 = 124.3 mm Hg; see Table 1-3) and within the first few minutes to hours of high-altitude exposure. The carotid body, sensing a decrease in PaO2, through a HIF-mediated process, signals the central respiratory center in the medulla to increase ventilation.153,281 This carotid body function (hypoxic ventilatory response [HVR]) is genetically determined562 but influenced by a number of extrinsic factors. Respiratory depressants such as alcohol and soporifics, as well as fragmented sleep, depress HVR. Agents that increase general metabolism, such as caffeine and coca, as well as specific respiratory stimulants, such as progesterone277 and almitrine,192 increase HVR. (Acetazolamide, a respiratory stimulant, acts on the central respiratory center rather than on the carotid body.) Physical conditioning apparently has no effect on HVR. Numerous studies have shown that a good ventilatory response enhances acclimatization and performance and that a very low HVR may contribute to illness433 (see Acute Mountain Sickness and High-Altitude Pulmonary Edema, later). However, over a normal range of values, HVR is not a reliable predictor of susceptibility to altitude illness.
Other factors influence ventilation on ascent to high altitude. As ventilation increases, hypocapnia produces alkalosis, which acts as a braking mechanism on the central respiratory center and limits a further increase in ventilation. To compensate for the alkalosis, within 24 to 48 hours of ascent the kidneys excrete bicarbonate, decreasing the pH toward normal; ventilation increases as the braking effect of the alkalosis is removed. Ventilation continues to increase slowly, reaching a maximum only after 4 to 7 days at the same altitude (see Figure 1-3). The plasma bicarbonate concentration continues to drop and ventilation to increase with each successive increase in altitude. Persons with lower oxygen saturation at altitude have higher serum bicarbonate values; whether the kidneys might be limiting acclimatization or whether this reflects poor respiratory drive is not clear.102 This process is greatly facilitated by acetazolamide (see Acetazolamide Prophylaxis, later).
The paramount importance of hyperventilation is readily apparent from the following calculation: the alveolar PO2 on the summit of Mt Everest (about 33 mm Hg) would be reached at only 5000 m (16,404 feet) if alveolar PCO2 stayed at 40 mm Hg, limiting an ascent without supplemental oxygen to near this altitude. Table 1-2 gives the measured arterial blood gas levels resulting from acclimatization to various altitudes.
Circulation
Systemic Circulation
Increased sympathetic activity on ascent causes an initial mild increase in blood pressure, moderate increases in heart rate and cardiac output, and increase in venous tone. Stroke volume is low because of decreased plasma volume, which drops as much as 12% over the first 24 hours577 as a result of the bicarbonate diuresis, a fluid shift from the intravascular space, and suppression of aldosterone.35 Resting heart rate returns to near sea level values with acclimatization, except at extremely high altitude. Maximum heart rate follows the decline in maximal oxygen uptake with increasing altitude. As the limits of hypoxic acclimatization are approached, maximum and resting heart rates converge. During Operation Everest II (OEII), cardiac function was appropriate for the level of work performed and cardiac output was not a limiting factor for performance.417,518 Interestingly, myocardial ischemia at high altitude has not been reported in healthy persons, despite extreme hypoxemia. This is partly because of reduction in myocardial oxygen demand from reduced maximal heart rate and cardiac output. Pulmonary capillary wedge pressure is low, and catheter studies have shown no evidence of left ventricular dysfunction or abnormal filling pressures in humans at rest.174,236 On echocardiography, the left ventricle is smaller than normal because of decreased stroke volume, whereas the right ventricle may become enlarged.518 The abrupt increase in pulmonary artery pressure can cause a change in left ventricular diastolic function, but because of compensatory increased atrial contraction, no overt diastolic dysfunction results.7 In trained athletes doing an ultramarathon, the strenuous exercise at high altitude did not result in left ventricular damage; however, wheezing, reversible pulmonary hypertension, and right ventricular dysfunction occurred in one-third of those completing the race and resolved within 24 hours.107
Pulmonary Circulation
On ascent to high altitude, a prompt but variable increase in pulmonary vascular resistance from hypoxic pulmonary vasoconstriction increases pulmonary artery pressure. Mild pulmonary hypertension is greatly augmented by exercise, with pulmonary pressure reaching near-systemic values,174 especially in persons with a previous history of high-altitude pulmonary edema.32,110 During OEII, Groves and colleagues174 demonstrated that even with a mean pulmonary artery pressure of 60 mm Hg, cardiac output remained appropriate and right atrial pressure did not rise above sea level values. Thus, right ventricular function was intact in spite of extreme hypoxemia and pulmonary hypertension in these well-acclimatized subjects.
Administration of oxygen does not completely restore pulmonary artery pressure to sea level values,324 likely because of vascular remodeling with medial hypertrophy. See Stenmark and associates509 for an excellent review of molecular and cellular mechanisms of the pulmonary vascular response to hypoxia, including remodeling. Pulmonary vascular resistance returns to normal within a few weeks after descent to low altitude.
Cerebral Circulation
Cerebral oxygen delivery, the product of arterial oxygen content and cerebral blood flow (CBF), depends on the net balance between hypoxic vasodilation and hypocapnia-induced vasoconstriction. CBF increases, despite hypocapnia, when PaO2 is less than 60 mm Hg (altitude greater than 2800 m [9186 feet]). In a classic study, CBF increased 24% on abrupt ascent to 3810 m (12,500 feet) and returned to normal over 3 to 5 days.491 These findings have been confirmed by positron emission tomography and brain magnetic resonance imaging (MRI) studies showing both elevations in CBF in hypoxia in humans and striking heterogeneity of the CBF, with CBF rising up to 33% in the hypothalamus and 20% in the thalamus, with other areas without significant changes.66,396 Cerebral autoregulation, the process by which cerebral perfusion is maintained as blood pressure varies, is impaired in hypoxia. Interestingly, this occurs both in newcomers, with acute246,296,521,547 and prolonged hypoxia,522 and in natives to high altitude.246 This observation raises questions about the importance of this process in AMS and acclimatization, because it appears to be a uniform response in all humans made hypoxemic. Overall, global cerebral metabolism seems well maintained with moderate hypoxia.103,356
Blood
Hematopoietic Responses to Altitude
Ever since the observation in 1890 by Viault550 that hemoglobin concentration was higher than normal in animals living in the Andes, scientists have regarded the hematopoietic response to increasing altitude as an important component of the acclimatization process. On the other hand, hemoglobin values apparently have no relationship to susceptibility to high-altitude illness.
In response to hypoxemia, erythropoietin is secreted by the kidneys and stimulates bone marrow production of red blood cells.483 The hormone is detectable within 2 hours of ascent, nucleated immature red blood cells can be found on a peripheral blood smear within days, and new red blood cells are in circulation within 4 to 5 days. Over a period of weeks to months, red blood cell mass increases in proportion to the degree of hypoxemia. Iron supplementation can be important; women who take supplemental iron at high altitude approach the hematocrit values of men at altitude202 (Figure 1-4).

FIGURE 1-4 Hematocrit changes on ascent to altitude in men and in women with and without supplemental iron.
(Modified from Hannon JP, Chinn KS, Shields JL: Effects of acute high altitude exposure on body fluids, Fed Proc 28:1178, 1969.)
The increase in hemoglobin seen 1 to 2 days after ascent is due to hemoconcentration secondary to decreased plasma volume, rather than a true increase in red blood cell mass. This results in a higher hemoglobin concentration at the cost of decreased blood volume, a trade-off that might impair exercise performance. Longer-term acclimatization leads to an increase in plasma volume as well as in red blood cell mass, thereby increasing total blood volume. Overshoot of the hematopoietic response causes excessive polycythemia, which may impair oxygen transport because of increased blood viscosity. Although the “ideal” hematocrit at high altitude is not established, phlebotomy is often recommended when hematocrit values exceed 60% to 65%. During the American Medical Research Expedition to Mt Everest (AMREE), hematocrit was reduced by hemodilution from 58% ± 1.3% to 50.5% ± 1.5% at 5400 m (17,717 feet) with no decrement in maximum oxygen uptake and increased cerebral functioning.465
Oxyhemoglobin Dissociation Curve
The oxygen dissociation curve (ODC) plays a crucial role in oxygen transport. The sigmoidal shape of the curve allows well-maintained SaO2 up to 3000 m (9843 feet), despite significant decreases in PaO2 (see Figure 1-1). Above 3000 m (9843 feet), small changes in PaO2 cause large changes in SaO2 (Figure 1-5). Because PaO2 determines diffusion of oxygen from capillary to cell, small changes in PaO2 can have clinically significant effects. This is often confusing for clinicians because SaO2 appears relatively well preserved. At high altitude, small changes in PaO2 lead to lower O2 uptake that can have a large effect on systemic hypoxemia, and thus on clinical status, while the SaO2 may appear relatively unchanged.
In 1936, Ansel Keys and colleagues266 demonstrated an in vitro right shift in position of the ODC at high altitude, favoring release of oxygen from blood to tissues. This change, due to increased 2,3-diphosphoglycerate, is proportional to the severity of hypoxemia. In vivo, however, the alkalosis at moderate altitude offsets this, and no net change occurs. In contrast, the marked alkalosis of extreme hyperventilation, as measured on the summit and simulated summit of Mt Everest (PaCO2 8 to 10 mm Hg, pH greater than 7.5), shifts the ODC to the left, facilitating oxygen-hemoglobin binding in the lung that raises SaO2 and is advantageous.462 Persons with a very left-shifted ODC, caused by an abnormal hemoglobin (Andrew-Minneapolis), when taken to moderate (3100 m [10,171 feet]) altitude, had less tachycardia and dyspnea and remarkably had no decrease in exercise performance.208 High-altitude adapted animals also have a left-shifted ODC.
Tissue Changes
The next link in the oxygen transport chain is tissue oxygen transfer, which depends on capillary perfusion, diffusion distance, and driving pressure of oxygen from the capillary to the cell. Banchero20 has shown that capillary density in dog skeletal muscle doubled in 3 weeks at a barometric pressure of 435 mm Hg. A recent study in humans noted no change in capillary density or in gene expression thought to enhance muscle vascularity.316 Ou and Tenney395 revealed a 40% increase in mitochondrial number but no change in mitochondrial size, whereas the study of Oelz and colleagues392 showed that high-altitude climbers had normal mitochondrial density. A significant drop in muscle size is often noted after high-altitude expeditions because of net energy deficit,316,319 resulting in increased capillary density and ratio of mitochondrial volume to contractile protein fraction as a result of the atrophy. Although there is no de novo synthesis of capillaries or mitochondria, the net result is a shortening of diffusion distance for oxygen.316,319
Sleep at High Altitude
Disturbed sleep is common at high altitude. There are multiple causes. Reflecting the great interest in sleep, more than 160 papers in the last 10 years have addressed sleep at altitude. The interested reader is referred to recent reviews.67,308,551,561,569 Nearly all subjects complain of disturbed sleep at high altitude, with severity increasing with the altitude. At moderate altitude, sleep architecture is changed, with a reduction in stages 3 and 4 sleep, stage 1 time increased, and little change in stage 2. Overall, there is a shift from deeper sleep to lighter sleep. In addition, more time is spent awake, with significantly increased arousals. Clinicians have reported either slightly less rapid eye movement (REM) time or no change in REM compared with what occurs at low altitude. REM sleep may improve over time at altitude.268 The subjective complaints of poor sleep are out of proportion to the small reduction (if any) in total sleep time and appear to be due to sleep fragmentation. With more extreme hypoxia, sleep time was dramatically shortened and arousals increased, without a change in ratio of sleep stages but with a reduction in REM sleep.10 The mechanisms of this change in sleep architecture and fragmentation are poorly understood. Periodic breathing appears to play only a minor role in altering sleep architecture at high altitude.461 The arousals have been linked to periodic breathing in some studies but not in others. Other factors might include change in circadian rhythm and perhaps body temperature.98 Obesity may explain susceptibility to both deranged sleep and sleep-disordered breathing in some individuals.155 Recent studies of infants and children590 and athletes in simulated altitude devices used for training also revealed deranged sleep quality in these groups.269,270,401 Although deranged sleep is a frequent complaint in high-altitude visitors, it seems to have little relation to susceptibility to altitude illness or other serious problems. Symptomatic treatment that avoids respiratory depression is safe (see Treatment under Acute Mountain Sickness, later).
Periodic Breathing
Periodic breathing is most common in early and light sleep, may occur during wakefulness when drowsy, and does not occur in REM sleep. The pattern is characterized by hyperpnea followed by apnea (Figure 1-6) and is caused by a battle for control of breathing between peripheral chemoreceptors (carotid body) and the central respiratory center. Respiratory alkalosis during hyperpnea acts on the central respiratory center, causing apnea. During apnea, SaO2 decreases, carbon dioxide increases, and the carotid body is stimulated, causing a recurrent hyperpnea and apnea cycle. The apnea is central, not associated with snoring, and occurs with absence of rib cage movement. Persons with high hypoxic ventilatory response have more periodic breathing,55 with mild oscillations in SaO2,282 whereas persons with low hypoxic ventilatory response have more regular breathing overall but may suffer periods of apnea with extreme hypoxemia distinct from periodic breathing.192 As acclimatization progresses, periodic breathing lessens but does not disappear, especially over 5000 m (16,404 feet), and sleep SaO2 increases (Figure 1-7; see Figure 1-6).10,55,524 Periodic breathing has not been implicated in the etiology of high-altitude illness, but nocturnal oxygen desaturation has been implicated.55,133,150 Eichenberger and colleagues130 have also reported greater periodic breathing in persons with HAPE, secondary to lower SaO2. As with fragmented sleep, intensity of periodic breathing is quite variable. Total sleep time with periodic breathing can vary from 1% to over 90%.598 Most studies report no association between periodic breathing and AMS.55 This may relate to the fact that persons with periodic breathing tend to have higher HVR and greater average ventilation and oxygenation.561
Pharmaceutical Aids
Acetazolamide, 125 mg at bedtime, diminishes periodic breathing and awakenings, improves oxygenation and sleep quality, and is a safe and superior agent to use as a sleeping aid (see Figure 1-6). It has the added benefit of diminishing symptoms of AMS. Other agents include diphenhydramine (Benadryl, 50 to 75 mg) or the short-acting benzodiazepines such as triazolam (Halcion, 0.125 to 0.25 mg) and temazepam (Restoril, 15 mg).308 Although caution is warranted for any agent that might reduce ventilation at high altitude, some studies have suggested that benzodiazepines in low doses are generally safe in this situation.124,164,384 Another option is to use both acetazolamide and a benzodiazepine. Bradwell and colleagues63 showed that acetazolamide (500 mg slow-release orally) given with temazepam (10 mg orally) improved sleep and maintained SaO2, counteracting a 20% decrease in SaO2 when temazepam was given alone. The nonbenzodiazepine hypnotic zolpidem (Ambien, 10 mg) was shown to improve sleep at 4000 m (13,123 feet) without adversely affecting ventilation.45
Exercise
Maximal oxygen consumption drops dramatically on ascent to high altitude.151,434 Maximal oxygen uptake () falls from sea level by approximately 10% for each 1000 m (3281 feet) of altitude gained above 1500 m (4921 feet). Persons with the highest sea level
values have the largest decrement in
at high altitude, but overall performance at high altitude is not consistently related to sea level
.392,428,566 In fact, many of the world’s elite mountaineers have quite average
values, in contrast with other endurance athletes.392 Acclimatization at moderate altitudes enhances submaximal endurance time but does not enhance
(Figure 1-8).151
After every few steps, we huddle over our ice axes, mouths agape, struggling for sufficient breath to keep our muscles going. I have the feeling I am about to burst apart. As we get higher, it becomes necessary to lie down to recover our breath.347
In contrast to the increase in ventilation with exercise, at increasing altitudes in OEII, cardiac function and cardiac output were maintained at or near sea level values for a given oxygen consumption (workload).417 Recent work has attributed the altitude-induced drop in to the lower PIO2, impairment of pulmonary gas exchange, and reduction of maximal cardiac output and peak leg blood flow, each explaining about one-third of the loss in
.76 However, mechanisms to explain the impairment of gas exchange and the lower blood flow remain elusive. Wagner556 proposes that the pressure gradient for diffusion of oxygen from capillaries to the working muscle cells may be inadequate. Another concept is that increased cerebral hypoxia from exercise-induced desaturation is the limiting factor.91,241,356,519,520 Mountaineers, for example, become light-headed and their vision dims when they move too quickly at extreme altitude (Figure 1-9).564
Training at High Altitude
Optimal training for increased performance at high altitude depends on the altitude of residence and the athletic event. For aerobic activities (events lasting more than 3 or 4 minutes) at altitudes above 2000 m (6562 feet), acclimatization for 10 to 20 days is necessary to maximize performance.104 For events occurring above 4000 m (13,123 feet), acclimatization at an intermediate altitude is recommended. Highly anaerobic events at intermediate altitudes require only arrival at the time of the event, although mountain sickness may become a problem.
The benefits of training at high altitude for subsequent performance at or near sea level depend on choosing the training altitude that maximizes the benefits and minimizes the “detraining” inevitable when maximal oxygen uptake is limited (altitude greater than 1500 to 2000 m [4921 to 6562 feet]). Hence, data from training above 2400 m (7874 feet) have shown no increase in subsequent sea level performance. Also, intermittent exposures to hypoxia seem to have no benefit.251,540 Runners returning to sea level after 10 days’ training at 2000 m (6562 feet) had faster running times and an increase in aerobic power, plasma volume, and hemoglobin concentration.19 Today the “live high–train low” approach pioneered by Levine and Stray-Gundersen294,513 has been adopted by many endurance athletes. The optimal dose for specific sports is still being worked out,570 but overall, endurance athletes believe and the science supports a small, but significant improvement in sea level performance after participating in a live high–train low training camp.514 The benefit appears to be due to enhanced erythropoietin production and increased red cell mass, which requires adequate iron stores and thus usually iron supplementation.328,443,512 Some individuals do not respond to live high–train low, perhaps related to genetic polymorphisms and the inability to increase erythropoietin levels sufficiently to raise red cell mass and thus increase oxygen-carrying capacity.79,247
High-Altitude Syndromes
High-altitude syndromes are illnesses attributed directly to hypobaric hypoxia, even if exact mechanisms are unclear. Clinically and pathophysiologically these syndromes overlap. Rapidity of onset of hypoxia is crucial; for example, sudden exposure to extreme altitude may result in death from acute hypoxia (asphyxia), whereas more gradual ascent to the same altitude may result in AMS or no illness at all. Where symptoms of acute hypoxia end and AMS begins is vague, as reflected in the classic experiments of Bert.54 In terms of altitude illness, the general concept of a spectrum of illness with a common underlying pathogenesis is well accepted and provides a useful framework for discussion. For the neurologic syndromes, the spectrum progresses from high-altitude headache to AMS to high-altitude cerebral edema (HACE). In the lungs, the spectrum includes pulmonary hypertension, interstitial edema, and HAPE. These problems all occur within the first few days of ascent to a higher altitude, have many common features, and respond to descent and/or oxygen. Longer-term problems of altitude exposure include high-altitude deterioration in sojourners and chronic mountain sickness in high-altitude residents.
Neurologic Syndromes
Neurologic syndromes reflect both nervous system sensitivity to hypoxia and effects of compensatory mechanisms. Although brain adenosine triphosphate (ATP) production and overall metabolism remain intact during moderate hypoxia, impaired synthesis of neurotransmitters can produce cerebral symptoms such as lassitude, malaise, and cognitive defects. Compensatory cerebral vasodilation at altitude appears to be the trigger for altitude headache and contributes to development of AMS and HACE. However, much of the pathophysiology of AMS and HACE remains a mystery. Focal neurologic deficits without cerebral edema are also difficult to explain; cerebral artery spasm and watershed hypoxia have both been invoked. Understanding the exact etiology of these neurologic syndromes will parallel advances in neuroscience; the emphasis in altitude illness is finally and appropriately on the brain.438
Acute Cerebral Hypoxia
But soon I was keeping absolutely motionless, without suspecting that perhaps I had already lost use of my movements. Towards 7500 m [24,606 feet], the numbness one experiences is extraordinary. The body and the mind weaken little by little, gradually, unconsciously, without one’s knowledge. One does not suffer at all; on the contrary. One experiences inner joy, as if it were an effect of the inundating flood of light. One becomes indifferent; one no longer thinks of the perilous situation or of the danger; one rises and is happy to rise. Vertigo of the lofty regions is not a vain word. But as far as I can judge by my personal impression, this vertigo appears at the last moment; it immediately precedes annihilation—sudden, unexpected, irresistible. I wanted to seize the oxygen tube, but could not raise my arm. My mind, however, was still very lucid. I was still looking at the barometer; my eyes were fixed on the needle which soon reached the pressure number of 280, beyond which it passed. I wanted to cry out “We are at 8,000 meters.” But my tongue was paralyzed. Suddenly I closed my eyes and fell inert, completely losing consciousness.54
There exists a parallelism to the smallest details between two animals, one of which is subjected in normal air to a progressive diminution of pressure to the point of death, while the other breathes, also to the point of death, under normal pressure, an air that grows weaker and weaker in oxygen. Both will die after having presented the same symptoms.54
Bert goes on to describe the symptoms of acute exposure to hypoxia:
It is the nervous system which reacts first. The sensation of fatigue, the weakening of the sense perceptions, the cerebral symptoms, vertigo, sleepiness, hallucinations, buzzing in the ears, dizziness, pricklings … are the signs of insufficient oxygenation of central and peripheral nervous organs. … The symptoms … disappear very quickly when the balloon descends from the higher altitudes, very quickly also … the normal proportion of oxygen reappears in the blood. There is an unfailing connection here.54
High-Altitude Headache
The term high-altitude headache (HAH) has been used in the literature for decades, and studies directed toward the pathophysiology and treatment of HAH have been reported. Obviously these are to an extent also studies of AMS. Headache lends itself to investigation better than do some other symptoms, because headache scores have been well validated.244 Headache is generally the first unpleasant symptom consequent to altitude exposure and is sometimes the only symptom.214 It may or may not be the harbinger of AMS, which is defined as the presence of headache plus at least one of four other symptoms, in the setting of an acute altitude gain.435 One could even argue that it is the headache itself that causes other symptoms such as anorexia, nausea, lassitude, and insomnia, as is commonly seen in migraine or tension headaches, and that mild AMS is essentially due to headache. The International Headache Society206 has defined high-altitude headache as headache with at least two of the following characteristics: (1) bilateral; (2) frontal or frontotemporal; (3) dull or pressing quality; (4) mild or moderate intensity; and (5) aggravated by exertion, movement, straining, coughing, or bending. In addition, the headache must occur with an ascent to altitude greater than 2500 m (8202 feet), develop within 24 hours after ascent, and resolve within 8 hours after descent.
Recent studies have attempted to characterize the clinical features and incidence of headache at altitude (Table 1-4). Silber and co-workers496 found that 50 of 60 trekkers (83%) in Nepal up to 5100 m (16,732 feet) developed at least one headache when over 3000 m (9843 feet). Older persons were less susceptible; women and those with headaches in daily life had more severe headaches, but no more headaches than others. Of those with headache, 52% did not have AMS by the Lake Louise criteria. The clinical features were widely variable. In general, the headaches were bilateral, generalized, dull, exacerbated by exertion or movement, often occurring at night, and resolved within 24 hours. Thus the headaches had some features of increased intracranial pressure (ICP). Persons with history of migraine did not have a higher incidence of headache. Various medications alleviated the headaches, especially mild ones, 70% of the time.
TABLE 1-4 Clinical Characteristics of Headache
Characteristic | Percent Reporting |
---|---|
Location | |
Global | 65.6 |
Frontal | 18.9 |
Hemi-cranial | 8.7 |
Occipital | 3.6 |
Change side | 3.2 |
Quality | |
Pulsatile-burst | 75.3 |
Pressing/tightening | 14.8 |
Burning | 5.1 |
Dull | 4.8 |
Evolution | |
Oscillate | 36.7 |
Disappear | 18.1 |
Diminish | 17.8 |
Increase | 10.4 |
Equal | 10.4 |
Break out in | 6.6 |
Elements Worsening | |
Exercise | 49.5 |
Sudden movements | 30.2 |
Valsalva maneuver | 13.8 |
Light | 6.5 |
Elements Relieving | |
Rest | 41.8 |
Analgesics | 39 |
Darkness | 10.2 |
Activity | 9 |
Concurrent Symptoms | |
Anorexia | 26.8 |
Irritability | 26.5 |
Nausea | 16.3 |
Vomiting | 9.7 |
Apathy | 7.1 |
Gastric | |
Malaise | 6.1 |
Somnolence | 5.7 |
Dysphasia | 1.8 |
Feelings | |
Pessimism | 33.2 |
Anxiety | 29.5 |
Fear | 23.4 |
Isolation | 11.2 |
Strangeness | 2.7 |
Modified from Serrano-Duenas M: High-altitude headache: A prospective study of its clinical characteristics, Cephalalgia 25:1110, 2005.
Serrano-Duenas489 also described in detail HAH in 98 climbers in the Andes. Persons with history of primary headache were excluded. He found the following characteristics: holocranial, 65.6%; pulsatile-burst type quality, 75.3%; oscillating evolution, 36.7%; increasing with exercise, 49.5%; relieved by rest, 41.8%; concurrent symptoms referred to: anorexia, 26.8%, irritability, 26.5%, and finally pessimism and anxiety feelings, 33.2% and 29.5%, respectively. Headaches were worse after a night’s sleep, more common in men, were of moderate intensity, and responded to analgesics.
In general, the literature suggests that HAH can be prevented by the use of nonsteroidal antiinflammatory drugs65,72,157 and acetaminophen204 as well as the drugs commonly used for prophylaxis of AMS, acetazolamide and dexamethasone. Some agents appear more effective than do others, with ibuprofen and aspirin apparently superior to naproxen.65,70,73 A serotonin agonist (sumatriptan, a 5-HT1 [serotonin type 1] receptor agonist) was reported to be effective for HAH prevention and/or treatment in some studies,69,243,544 but not in others.28 Flunarizine, a specific calcium antagonist used for treatment of migraine, was not effective in one study.44 Interestingly, oxygen is often immediately effective for HAH (within 20 minutes) in subjects with and without AMS, indicating a rapidly reversible mechanism of the headache.26,191
The response to many different agents might reflect multiple components of the pathophysiology, or merely the nonspecific nature of analgesics. As Sanchez del Rio and Moskowitz463 have pointed out, different inciting factors for headache may result in a final common pathway, such that the response to different therapies is not necessarily related to the initial cause of the headache. They have provided a useful multifactorial concept of the pathogenesis of HAH, based on current understanding of headaches in general.463 They suggest that the trigeminovascular system is activated at altitude by both mechanical and chemical stimuli (vasodilation, nitric oxide [NO] and other noxious agents), and in addition, the threshold for pain is likely altered at high altitude (Figure 1-10).463 If AMS and especially HACE ensue, then altered intracranial dynamics may also play a role, via compression or distension of pain-sensitive structures. A recent study provided evidence against sustained trigeminovascular activity,14 but more study will hopefully bring better understanding of the pathophysiology of these often debilitating headaches, and new treatments as well.
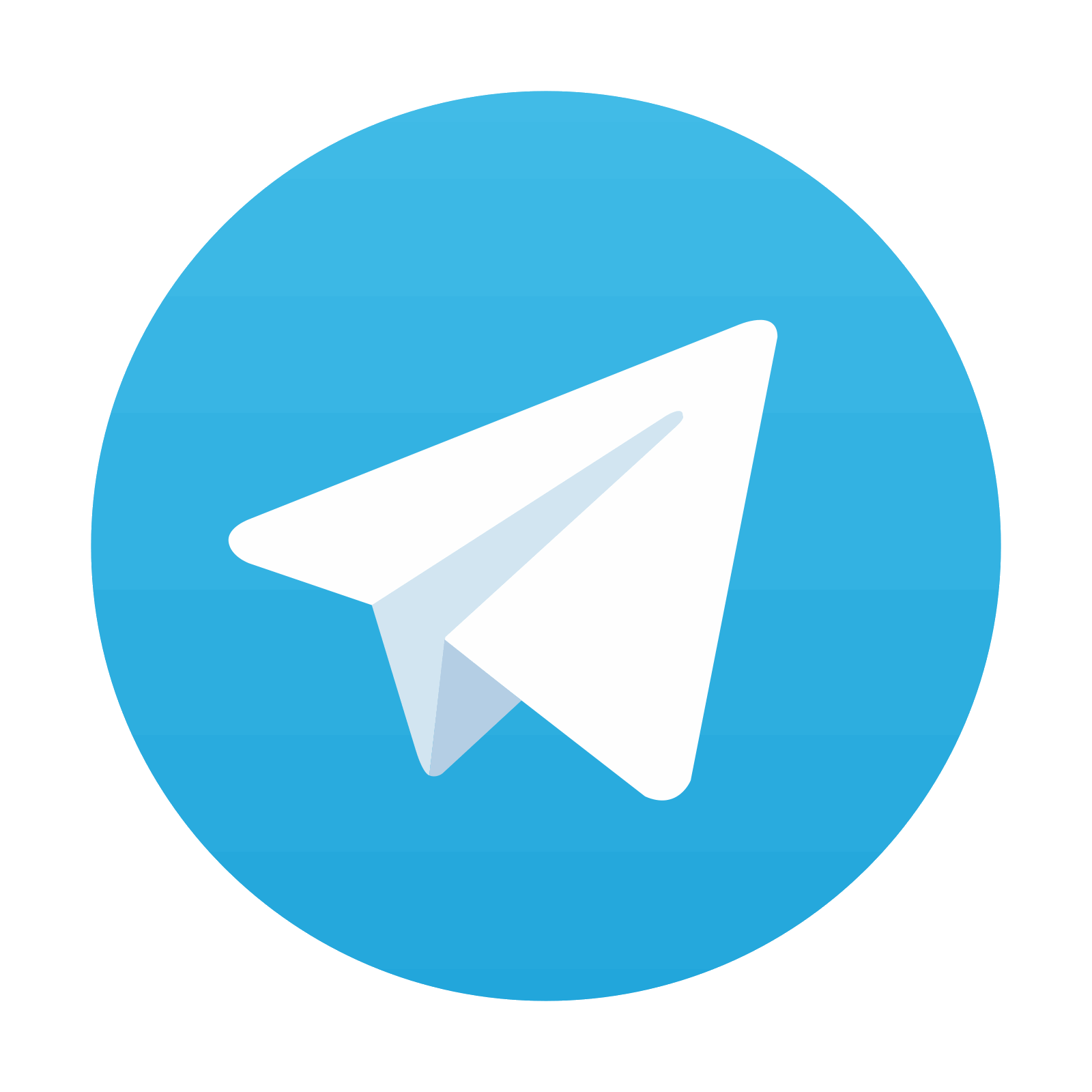
Stay updated, free articles. Join our Telegram channel
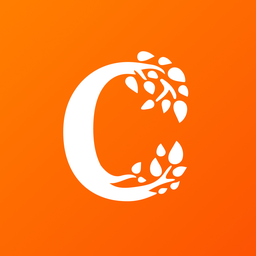
Full access? Get Clinical Tree
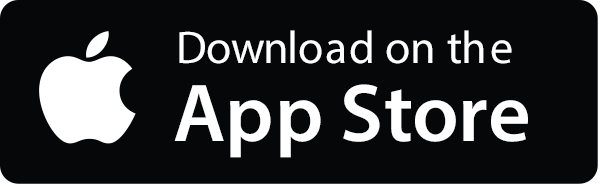
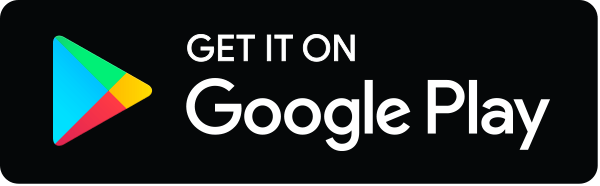