Felice Eugenio Agrò, Chiara Piliego Managing fluid administration during lung resection by the anesthesiologist is very similar to a tightrope walker, trying to balance the risk of acute lung injury and of acute kidney injury. There is a narrow interval between fluid overload and hypovolemia, two conditions that may impair lung and renal function, respectively. This challenge is crucial to win because it has a profound impact on perioperative morbidity and mortality. The aim of this chapter is to discuss how much and which fluid to administer, trying to guide fluid management. fluid management; lung resection; acute lung injury; acute kidney injury; perioperative care Managing fluid administration during lung resection by the anesthesiologist is very similar to a tightrope walker trying to balance the risk of acute lung injury (ALI) and that of acute kidney injury (AKI); there is a narrow margin of safety between fluid overload and hypovolemia, two conditions that may impair lung and renal function, respectively. Maintaining fluid and electrolyte homeostasis represents a crucial challenge because of the profound impact on perioperative morbidity and mortality. The evolution in surgical techniques lead to reduction of in-hospital mortality rates to less than 2% for lobectomies and less than 6% for pneumonectomies.1,2 The length of hospital stay is also shorter, and with cumulative experience, procedural complications are less frequent. The main causes of mortality are not from surgical or cardiac complications, but rather being replaced by pulmonary pathology, such as pneumonia, empyema, and ALI.3 Pulmonary complications are caused by a combination of multiple factors acting together and among them, the volume of intravenous fluids administered intraoperatively plays an important role.4 The aim of this chapter is to provide a guide for fluid management during lung resection, answering some question, yet raising some others. Postpneumonectomy pulmonary edema (PPPE) was first reported by Zeldin, who described the correlation between the amount of perioperative fluid, over 37 mL/kg intraoperatively and for the first 24 hours after surgery, and pulmonary complications.5 This article laid the foundations for establishing an association between fluid overload and development of lung injury but has some issues to consider. First, the edema affected only 10 patients, which makes the sample size low for current standards. Most of the PPPE occurred in patients who had right pneumonectomies, which has a larger parenchymal tissue. These pulmonary complications can be present not only in pneumonectomies, but also after less extensive resections or even after thoracotomies without resections.6 However, after Zeldin, several investigators attempted to explore the correlation between fluid administration and lung outcome.7–10 Licker et al.11 in a multivariate analysis, described two independent risk factors for ALI: the extent of lung resection (pneumonectomy was associated to the highest risk) and fluid overload (meaning 9.1 mL/kg/h intraoperatively and 1 mL/kg/h for the first 24 hours). This study however, did not provide for protective lung ventilation (PLV): a latter comparison with a group of patients with adoption of PLV showed a pronounced decrease in the incidence of ALI/acute respiratory distress syndrome (ARDS), despite no change in fluid administration. This finding suggests the importance of ventilation strategy in lung outcomes. However, some studies report the presence of lung injury even when applying a “very restrictive ventilatory strategy.”12 Postoperative lung injury usually occurs 1 to 4 days after surgery, and presents with a low pulmonary capillary wedge pressure, which raises questions if the fluid overload is the sole accountable factor. Certainly, this measure confirms that this type of edema has not its origin from heart failure, but—au contraire—it challenges the hypothesis of hypervolemia. In addition, the edema fluid has high concentrations of proteins.13 This type of pulmonary edema seems to be the result of an increase in pulmonary capillary permeability, associated with a rise in plasma protein content; it suggests that this lung injury could occur despite normovolemia, and most likely is being just exacerbated by fluid overload. The clinical pattern formerly known as PPPE shares many clinical and histologic features with ARDS. It is unclear which studies are referring to ALI or postoperative lung injury rather than PPPE. The definition of ALI and ARDS, according to the American-European Consensus Conference on ARDS,14 consists of a syndrome of inflammation and increased permeability with: This definition can be applied to postthoracotomy ALI, even if there is a broad spectrum of clinical pictures described in patients with postthoracotomy lung injury.15 Lung injury following lung resection occurs in 2.5% of all lung resections, with increased incidence of 7.9% after pneumonectomy.16 Historically, the incidence of kidney damage in thoracic surgery was reported to be quite low. The Society of Thoracic Surgeons database cited an overall incidence of 1.4%.2 These data pertained only to patients who required renal suppurative replacement therapy, and a subsequent assessment using standardized criteria reported a much higher incidence. In 2002, the Acute Dialysis Quality Initiative Group presented a classification system for AKI named RIFLE (risk, injury, failure, loss, and end-stage renal disease), based on increased serum creatinine (SCr) associated with a decrease in glomerular filtration rate (GFR) and urinary output.17 A more recent system was reported, called AKIN (Acute Kidney Injury Network) criteria:18 this system is considering an absolute increase in SCr of 0.3 mg/dL as a diagnostic criteria in identifying stage 1 disease. These criteria attracted much criticism by clinicians, questioning the clinical relevance of a minimal increase in SCr. However, Basile et al.19 showed worse outcomes in patients with postoperative AKI, even with slight increases of SCr and with complete restoration of kidney function; postthoracotomy AKI, specifically, was associated with a hazard ratio of 1.6 in terms of long-term survival. Studies using the AKIN criteria report a higher incidence of kidney injury. Ishikawa et al. reported an incidence of renal impairment in 5.9% of thoracic surgical patients,20 and Hobson reached 33% of incidence in a high-risk group.21 The classification systems are summarized in Tables 21.1 and 21.2. Table 21.1 GFR, Glomerular filtration rate; SCr, serum creatinine. Table 21.2 GFR, Glomerular filtration rate; SCr, serum creatinine. Ventilation can cause a broad spectrum of local and systemic pathophysiologic changes, in particular in patients requiring lung surgery and one-lung ventilation (OLV), defined as ventilator-induced lung injury (VILI). The causes of these adverse effects are barotrauma (direct consequence of high pressure on the lung), volutrauma (effect of excessive distension of the lung), atelectotrauma (cyclic opening and closing of alveoli) and biotrauma (inflammatory cascades triggered by cytokines release).22,23 Transfusion-related to acute lung injury (TRALI) diagnosis was classically based upon development of a new ALI within 6 hours of transfusion, not ascribable to another ALI risk factor.24 Recently, an updated TRALI definition/classification scheme has been published with several modifications. Among them is the distinction between two types of TRALI that should be noted TRALI type I applies to patients without an ARDS risk factor, TRALI type II to cases with an ARDS risk factor or even with mild preexisting ARDS. At first glance, one should immediately notice that this second type is in contrast with the old definition. This new diagnostic criteria for TRALI is listed in Box 21.1.25 Understanding the two main mechanisms that play a crucial role in the development of pulmonary edema following thoracic surgery, which are an increase in pulmonary capillary hydrostatic pressure and/or an increase in capillary permeability, is essential to improve patient outcomes. In 1896, Ernest Starling working on an animal model, stated that the mechanism of fluid exchange (a combination of filtration and reabsorption between capillaries and interstitial space) was found in differences between hydrostatic and osmotic pressures, as well as in the capillary wall structure.26 This observation led to the formulation of Starling equation as follows: Jv/A = Lp [(Pc – Pi) – σ (πc – πi)] Likewise Jv / A is the rate of fluid exchange per unit area of the vessel, Lp is the permeability of the vessel wall, (Pc – Pi) and (πc – πi) with a difference between capillary and interstitial fluid in terms of hydrostatic and osmotic pressure, and σ is the reflection coefficient of the vessel wall to plasma proteins. This equation depicts fluid exchange as a balance between opposing forces with capillary hydrostatic pressure representing the filtration force and the capillary osmotic pressure representing the reabsorption force; the larger is the difference, the greater is the exchange (Fig. 21.1). Pulmonary circulation is endowed with the ability to respond to significant variations in cardiac output without raising its capillary pressure, thanks to capillary recruitment mechanisms.27 This system maintains stable pulmonary pressure even if the left atrial pressure is doubled and pulmonary capillary filtration remains unvaried.28 Only when adaptive mechanisms are overcome, fluids start to collect in the interstitium. Because of the low compliance of this compartment and owing to the role of lymphatic drainage, there is an initial limitation to the accumulation of interstitial fluid. This protection cannot last long, because of fragmentation of the proteoglycan network of the interstitial matrix.29 The last protective mechanism is represented by the alveolar epithelium clearance of alveolar fluid is carried on through the epithelial sodium channels, stimulated by beta-adrenergic agonists30 (which are gaining attention as therapeutic agents in this field) and inhibited by endothelin-1.31 The Starling equation has some limitations different studies described deviations from it, both in vitro and in vivo32 (Fig. 21.2). These studies focused on the role of non-Starling mechanisms of barrier regulation, which involve the endothelium and more specifically, the endothelial glycocalyx layer (EGL).33 Fluids cross capillary endothelium through breaks in tight junctions, vesicles and leaky junctions, allowing the transport of molecules of various dimensions. EGL is a layer of proteoglycans and glycoprotein bound to the membrane, covering the luminal surface of vascular endothelium, with function of molecular barrier. It is a complex mesh of glycosaminoglycans and proteins, which filters circulating cells and macromolecules, so that they cannot enter the interstitium. The composition and dimensions of the glycocalyx fluctuate as it continuously replaces material sheared by flowing plasma.34 This brings us to the so-called revised Starling equation, which takes the EGL role in capillary fluid dynamics in considerations; the role it plays is carried out in two different functions. First, it represents a strainer for plasmatic proteins, altering the balance in oncotic pressure between plasma and interstitium as predicted by the traditional Starling’s equation.35 Secondly, it responds to fluid shear stress as a mechanosensory, eliciting an increase in capillary permeability in response to a raised capillary blood flow.36 It is easy to understand how important this structure is in maintaining fluid homeostasis, and, consequently, how deleterious its injury can be; to identify what can cause this injury, it is essential to completely understand the mechanisms of ALI. Loss of integrity in EGL can derive from multiple factors, for instance, inflammatory cytokines release,37 surgical trauma, and ischemia-reperfusion injury.38 In this framework, hypervolemia plays a major role exacerbating dilution of plasma proteins and atrial natriuretic peptide release. This causes an increase in vessel permeability, with consequent extravasation of fluid and exposition of leucocyte adhesion molecules (which fosters inflammation), and triggers a vicious cycle, inevitably leading to ALI.39 Some colloids do appear to be markedly superior as resuscitation fluids expansion because they preserve the glycocalyx. In hemorrhagic shock, for example, resuscitation with higher ratios of plasma seems to result in lower mortality than crystalloid, despite there being seemingly little advantage in terms of preservation of coagulation factors. The explanation may lie not with the Starling equation but rather that certain colloids act to preserve the endothelial glycocalyx. Multiple risk factors seem to be involved in this clinical pattern development and are summarized in Box 21.2. Rather than a single risk factor being responsible for ALI, it is likely that a combination of multiple-hit series of damages harms the alveolar epithelial and capillary endothelium, as well as extracellular matrix.40 In ARDS, the multiple-hit hypothesis presents several pathophysiologic injuries, which may not cause lung damage when presented separately, but, when collected, result in the clinical syndrome of ALI or ARDS. In postthoracotomy ALI, the “first hit” is represented by an activation of the systemic inflammatory response (because of surgical trauma, manipulation, or atelectasis).41 Among the possible “second hits” we can find all the risk factors described before, which aggravates the injury and results in increasing lung insult. Alam et al. conducted a retrospective case-control study of consecutive patients undergoing resection for lung cancer. The severity of lung injury was defined using the American European Consensus Conference on ARDS. Patients with lung injury were compared with matched control patients, based on age, sex, and extent of resection, for examination of a priori defined risk factors. Some 1428 patients underwent lung cancer resection. Postoperative lung injury occurred in 76 (5.3%) cases, 44 (3.1%) of which met criteria for ARDS. After univariate and multivariate analysis, increasing perioperative fluid administration and decreasing postoperative predicted lung function were significant risk factors for the development of lung injury. The overall mortality for patients with lung injury was 25%, compared with 2.6% for the control group. They concluded that the odds ratio of developing ALI after lung resection of 1.17 for every 500 mL increase in perioperative fluid administration.42 Several studies and meta analyses demonstrated that fluid overload is constantly related to postoperative lung injury; this led to the adoption of restrictive fluid management. On the other hand, recent literature encourage an optimal strategy for fluid management, rather than towards a restrictive one. Excessive fluid administrations are not only responsible for postthoracotomy ALI, they is important anesthetic- and surgery-related risk factors. With fluids “we can make the lung injury worse, but we do not cause it.”43 As is the case for lung, the kidney is insulted by a multifactorial process in this clinical setting. It is well known that anesthetic drugs generally impair renal function, decreasing GFR, urinary output, and sodium excretion,44 and that surgery may sometimes unmask latent kidney disease often underlying, hypertension-, diabetes- or atherosclerosis-related. Thoracic surgery triggers a systemic inflammatory response that may result in kidney injury. Hypervolemia as well as hypovolemia may be related to the appearance of AKI. Hypervolemia increases the kidney workload to maintain fluid clearance. Hobson et al. investigated the incidence of AKI after thoracic surgery, and described its association with larger amounts of crystalloid administration.21 However, patients who received higher volumes had longer operative times, and it is unclear which factor caused the worsening of kidney function. On the other hand, hypotension because of hypovolemia causes decreased renal perfusion, which when associated with the surgery-related inflammatory state, may promote the development of AKI.45,46 The role of mechanical ventilation worsening capillary and (subsequently) lung damage has to be briefly presented. Gattinoni et al.47 demonstrated the role of high tidal volumes used during mechanical ventilation of healthy lungs. High tidal volumes, indeed, cause barotraumas, stress ruptures because of the excessive stretching of the alveolar maximal physiologic strain is overtaken, beyond total lung capacity. The tidal volume ventilation of a patient with ARDS can causes stress rupture and should be reduced to protect the lung. This consideration represents the foundation for the concept of “baby lung”, which exposes lungs to a greater risk for VILI. This concept originates from the analysis of computed tomography lung scans of adult patients with severe ARDS: the images showed an amount of aerated lung of only 200 to 500 g, that corresponds to the lung tissue of a 6-year-old child.48 Thus OLV has even more adverse effects.49 It has been demonstrated that by applying two-lung ventilation tidal volume to a single lung ventilation, the peak inspiratory pressure will rise to about 55% and plateau pressure increases to 42%. Peak inspiratory pressure is dependent on tidal volume, inspiratory time, endotracheal size, and presence or absence of bronchospasm. It does not represent the distending pressure transmitted to the alveoli, meaning it is not necessarily the actual cause of alveolar trauma. Plateau pressure elevation, on the other hand, is the real culprit of the lung damage, reflecting the actual distention pressure of the alveoli. No threshold has been identified to be actually safe. Plateau pressure is inherently related to lung volume; analyzing the physiopathology of VILI, this is an important consideration because it helps to understand that barotrauma and volutrauma proceed at the same pace (or, better that increase of the plateau pressure proceeds at the same pace to create lung distension). Stress strain inducing deformation causes rapid lipid transport to the plasma membrane, to increase the surface area, prevent membrane disruption and repair the damaged cells.50 When these protective mechanisms are overtaken, though, cells are detached from the basement membrane, cell junctions in epithelium and endothelium are smashed losing the tight junction between the cells, and the formation of intracapillary blebs, alveolar, will initiate the formation of interstitial edema.51 Protective ventilation strategies are applied in an attempt to protect the lung from barotrauma, limiting plateau pressures, as well as from volutrauma, avoiding overdistension. In ARDS, both increased weight of the edematous lung and surfactant dysfunction are involved in regional atelectasis; atelectatic lung units are still recruitable, so that during ventilation, cyclic opening and collapse of these units occur.52 Recruitment at the interface air-collapsed airway causes mechanical injuries to the atelectasis alveoli. The flooded alveoli that are responsible for interfacial stress, disrupting the plasma membrane-cytoskeleton adhesion, results in formation and destruction of foam bubbles at the gas-liquid interface. Although the exact nature of airway/alveolar “closure” might include both compliant collapse and/or the flooding of noncollapsed airways, in either case, airway “reopening” involves the movement of an air-liquid interface or a microbubble of air that displaces the surrounding fluid. As shown schematically (in Fig. 21.3) these “microbubble” flows will result in the application of a complex, nonuniform, and transient hydrodynamic stress field on the epithelial cell lining the airway/alveolar walls.53,54 Microbubble flows exert complex fluid mechanical forces on the airway wall. The schematic diagrams of airway reopening in a noncollapsed fluid-filled airway forces, shown schematically as arrows, include normal pressure and tangential shear stresses, as well as temporal and spatial gradients in these stresses. Hypothetical cell deformations because of these stresses at different locations are shown schematically in the circular inserts.53 Repetitive inflation/deflation cycles of lung units are therefore involved in the multifactorial genesis of perioperative ALI. Lastly, in response to mechanical lung injury, a systemic biologic cascade is triggered, releasing proinflammatory and proinjurious cytokines. This response may promote damage in lung regions even when not initially influenced by mechanical insult, and also spread extrapulmonary organ injury, predisposing to multiorgan failure.55 This pathway, named biotrauma, may not seem so significant. It should be remembered, though, that the epithelial surface area of one single adult lung is about 60 to 80 square meters, so that the release of proinjurious mediators is amplified.56 In addition, the blood flow through the pulmonary circulation is substantial, so that any mediator produced by the lung is rapidly transported with the blood stream throughout the whole body. In conclusion, referring to the multiple hit hypothesis, VILI (promoted by the combination of barotrauma, volutrauma, atelectotrauma, and biotrauma) represents a first hit. In thoracic surgery in particular, OLV and surgical manipulation should be considered as the second hit, and the third hit may result from reexpansion/reperfusion lung injury.57
Fluid Management During Lung Resection
Abstract
Keywords
Introduction
From Postpneumonectomy Pulmonary Edema to Acute Lung Injury
Definitions and Epidemiology
Postthoracotomy Acute Lung Injury
Acute Kidney Insufficiency
Stage
Glomerular Filtration Rate
Urinary Output
Risk
↑SCr × 1.5 or ↓GFR >25%
<0.5 mL/kg/h for 6 hours
Injury
↑SCr × 2 or ↓GFR >50%
<0.5 mL/kg/h for 12 hours
Failure
↑SCr × 3 or ↓GFR >75% or SCr 4 mg/dL with acute rise of 0.5
<0.3 mL/kg/h for 24 hours or anuria >12 hours
Loss
Complete loss of kidney function for > 4 weeks
End-stage
End-stage kidney disease >3 months
Stage
Creatinine
Urinary Output
I
↑SCr × 1.5–2 or ↑SCr 0.3 mg/dL
<0.5 mL/kg/h for 6 hours
II
↑SCr × 2–3
<0.5 mL/kg/h for 12 hours
III
↑SCr × 3 or ↓GFR >75% or SCr 4 mg/dL with acute rise of 0.5 or any dialysis
<0.3 mL/kg/h for 24 hours or anuria >12 hours
Ventilator-Induced Lung Injury
Transfusion-Related to Acute Lung Injury
Physiopathology
Acute Lung Injury
Starling Forces
Hydrostatic Edema
Revised Starling and Role of Endothelial Glycocalyx Layer
Risk Factors for Acute Lung Injury and Multiple-Hit Hypothesis
The Role of Fluid Overload
Acute Kidney Injury
Ventilator-Induced Lung Injury
Stay updated, free articles. Join our Telegram channel
Full access? Get Clinical Tree
Fluid Management During Lung Resection
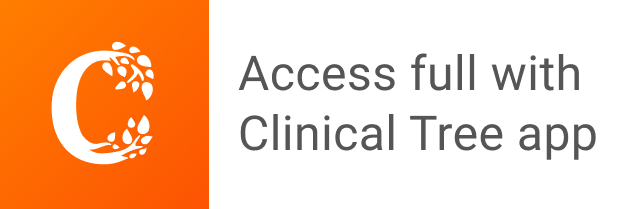