Fluid, Electrolyte and Acid–Base Balance
COMPARTMENTAL DISTRIBUTION OF TOTAL BODY WATER
The distribution of TBW between the main body compartments is illustrated in Figure 12.1. One-third of TBW is contained in the extracellular fluid volume (ECFV) and two-thirds in the intracellular fluid volume (ICFV). The ECFV is subdivided further into the interstitial and intravascular compartments. In addition to the absolute volumes of each compartment, Figure 12.1 shows the relative size of each compartment compared with body weight.
SOLUTE COMPOSITION OF BODY FLUID COMPARTMENTS
Intracellular Fluid
Figure 12.2 shows the solute composition of the main body fluid compartments. Although the total concentration of intracellular ions exceeds that of extracellular ions, the numbers of osmotically active particles (and thus the osmolalities) are the same on each side of the cell membrane (290 mosmol kg–1 of solution).
WATER HOMEOSTASIS
The principal sources of body water are ingested fluid, water present in solid food and water produced as an end-product of metabolism. Intravenous fluids are another common source in hospital patients. Actual and potential outlets for water are classified conventionally as sensible and insensible losses. Insensible losses emanate from the skin and lungs; sensible losses occur mainly from the kidneys and gastrointestinal tract. Figure 12.3 depicts the daily water balance in a 70 kg adult in whom input and output balance. It should be noted that sources of potential loss are not evident in this diagram. For example, over 5 L of fluid are secreted daily into the gut in the form of saliva, bile, gastric juices and succus entericus, yet only 100 mL of fluid is present in faeces. This illustrates the potential that exists for significant fluid loss in the presence of disease.
PRACTICAL FLUID BALANCE
Table 12.1 shows the electrolyte contents of five intravenous solutions used commonly in the United Kingdom. These solutions are adequate for most clinical situations. Two self-evident but important generalizations may be made regarding solutions for intravenous infusion.
Rule 1: All infused Na+ remains in the ECF; Na+ cannot gain access to the ICF because of the sodium pump. Thus, if saline 0.9% is infused, all Na+ remains in the ECF. As this is an isotonic solution, there is no change in ECF osmolality and therefore no water exchange occurs across the cell membrane. Thus, saline 0.9% expands ECFV only. However, if saline 0.45% is given, ECF osmolality decreases; this causes a shift of water from ECF to ICF. If saline 1.8% is administered, all Na+ remains in the ECF, its osmolality increases and water moves from ICF to ECF to maintain osmotic equality.
Rule 2: Water without sodium expands the TBW. After infusion of a solution of glucose 5%, the glucose enters cells and is metabolized. The infused water enters both ICF and ECF in proportion to their initial volumes.
Table 12.2 illustrates the results of infusion of 1 L of saline 0.9%, saline 0.45% or glucose 5% in a 70 kg adult.
TABLE 12.2
Compartmental Expansion Resulting from Infusion of 1 L of Saline 0.9%, Saline 0.45% or Glucose 5%
Assessment of daily fluid requirements may be allocated usefully into three processes:
Normal Maintenance Needs
Water. Regardless of the disease process, water and electrolyte losses occur in urine and as evaporative losses from skin and lungs. It is evident from Figure 12.3 that a normothermic 70 kg patient with a normal metabolic rate may lose 2500 mL of water per day. Allowing for a gain of 400 mL from water of metabolism, this hypothetical patient needs about 2000 mL day–1 of water. As a rule of thumb, a volume of 30–35 mL kg–1 day–1 of water is a useful estimate for daily maintenance needs.
Sodium. The normal requirement is 1 mmol kg–1 day–1 (50–80 mmol day–1) for adults.
Potassium. The normal requirement is 1 mmol kg–1 day–1 (50–80 mmol day–1) for adults.
Existing Deficits
Dehydration with accompanying salt loss is a common disorder in the acutely ill surgical patient.
Assessment of Dehydration
This is a clinical assessment based upon the following.
mild: loss of 4% body weight (approximately 3 L in a 70 kg patient) – reduced skin turgor, sunken eyes, dry mucous membranes
moderate: loss of 5–8% body weight (approximately 4–6 L in a 70 kg patient) – oliguria, orthostatic hypotension and tachycardia in addition to the above
severe: loss of 8–10% body weight (approximately 7 L in a 70 kg patient) – profound oliguria and compromised cardiovascular function.
Perioperative Fluid Therapy
if blood or serum is lost from drains (colloid solutions should be used if losses exceed 500 mL)
if gastrointestinal losses continue, e.g. from a nasogastric tube or a fistula
after major surgery (e.g. oesophagectomy, total gastrectomy, aortic aneurysm repair), when additional water and electrolytes may be required for 24–48 h to replace continuing third-space losses
during rewarming if the patient has become hypothermic during surgery.
After major surgery, assessment of fluid and electrolyte requirements is achieved best by measurement of CVP and serum electrolyte concentrations. Fluid and electrolyte requirements in infants and small children differ from those in the adult (see Ch 36).
SODIUM AND POTASSIUM
Disorders of Sodium/Water Balance
Hypernatraemia is defined as a plasma sodium concentration of more than 150 mmol L–1 and may result from pure water loss, hypotonic fluid loss or salt gain. In the first two conditions, ECFV is reduced, whereas salt gain is associated with an expanded ECFV. For this reason, the clinical assessment of volaemic status is important in the diagnosis and management of hypernatraemic states. The common causes of hypernatraemia are summarized in Table 12.3. The abnormality common to all hypernatraemic states is intracellular dehydration secondary to ECF hyperosmolality. Primary water loss resulting in hypernatraemia may occur during prolonged fever, hyperventilation or severe exercise in hot, dry climates. However, a more common cause is the renal water loss that occurs when there is a defect in either the production or release of ADH (cranial diabetes insipidus) or an abnormality in response to ADH (nephrogenic diabetes insipidus).
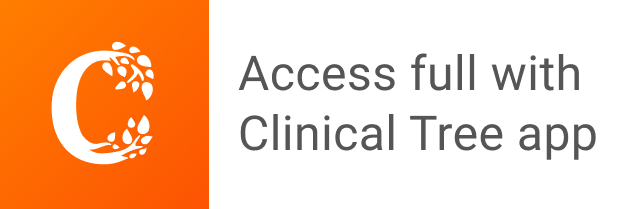