Fig. 7.1
Fluid compartments in the human body
Table 7.1
Electrolyte concentrations, plasma and intracellular
Ion | Plasma (meq/l) | Intracellular (meq/l) |
---|---|---|
Sodium | 140 | 12 |
Potassium | 4 | 150 |
Calcium | 5 | 1 |
Magnesium | 2 | 40 |
Chloride | 105 | 4 |
Bicarbonate | 24 | 7 |
Electrolytes are readily exchanged between intracellular and extracellular fluids in order to support cell metabolism. A membrane-bound ATP-dependent pump exchanges sodium for potassium in a ratio of 3:2. Intravascular pressure continuously forces fluid toward the interstitial space, but the equilibrium is maintained by the oncotic pressure created by large molecules, such as proteins and colloids. These large molecules cannot cross the barrier between compartments and thus maintain intravascular volume, thereby effectively maintaining circulation. Tonicity of a solution is usually expressed in terms of osmolarity (number of osmoles/liter of solution) or osmolality (number of osmoles/kg of solution). For nondissociative substances an osmole equals 1 mole, and for dissociative (ionized) substances, each mole results in “n” moles (e.g., NaCl produces 2 osmoles). A mole of a substance equals 6.02 × 1023 molecules. Normal plasma osmolality is 280–290 mosm/kg and can be calculated by the following formula:


Perioperative Fluid Management
The practice of perioperative fluid therapy has been under scrutiny for the past 50–60 years. In the late 1950s–1960s, two men began the debate with Francis Moore first arguing for a more restricted regimen, while Tom Shires supported a liberal administration of fluid. The latter’s theory was then supported during the Korean War where large amounts of fluid were infused to trauma patients resulting in improved survival. Tom Shires’ recommendation for excessive administration of fluids was then a common practice for decades, until the past 5–10 years where the deleterious effects of such management have been illustrated in the literature and a new approach to perioperative fluid management has been developed.
Hypovolemia
Hypovolemia can result from prolonged preoperative fasting, vasodilation caused by anesthesia drugs or neuraxial blockade, or acute bleeding. To understand when and how to manage fluid therapy, it is important to distinguish dehydration from intravascular hypovolemia. Dehydration affects the extravascular space and is a result from urine production and perspiration. This leads to a colloid-free fluid loss from the extracellular space and is treated by administering crystalloid solution. On the other hand, acute hypovolemia results from a loss of colloid-rich solution (plasma) from the intravascular space which lowers oncotic pressure and leads to interstitial edema. Using crystalloids to replace these losses may exacerbate the already low oncotic pressure and worsen edema. Therefore, using colloid solutions may be preferable when treating acute hypovolemia.
Hypervolemia
Various negative effects of excessive administration of fluid before, during, and after surgery have been well illustrated. Marked hypervolemia is defined as weight gain of at least 10 % over the preoperative weight. When volume overloading, there is the potential to impair left ventricular stroke volume or cause myocardial ischemia. Pulmonary function can also be impaired with accumulation of fluid in the interstitium leading to pulmonary edema, atelectasis, pneumonia, or respiratory distress. Furthermore, volume overload can lead to impaired tissue oxygenation resulting in impaired wound healing. Paralytic ileus may be worsened by excessive fluid administration. Finally, liberal fluid management can exacerbate the sodium and water-conserving effects of the surgical stress response and can result in electrolyte and acid–base disturbances.
The source of these side effects has been credited to various physiological responses within the body. Excessive fluid administration leads to shifting a patient along their Frank–Starling performance curve to the extreme right, leading to left ventricular strain, a decrease in stroke volume, and thus low cardiac output (Fig. 7.2). The myocardial dysfunction can result in pulmonary or peripheral edema and decreased oxygen delivery. Another aspect of volume overload leading to system dysfunction arises from “third spacing,” which results from fluid seeping from the intravascular space. This is believed to result from damage to two different layers of the endothelium—the endothelial surface layer (EDL) and the overlying glycocalyx. The EDL acts as a barrier between the intravascular space and the interstitial space. Positive hydrostatic pressure continuously forces fluid toward the interstitium. However, the glycocalyx contains large proteins which cannot cross the EDL, effectively increasing the oncotic pressure and keeping the majority of fluid within the intravascular space. Unfortunately, many factors such as surgical stress, ischemia/reperfusion injury, and circulating mediators such as TNF-alpha, cytokines, proteases, and atrial natriuretic peptide lead to thinning of the EDL and breakdown of the glycocalyx, and thus worsening the edema.


Fig. 7.2
Frank–Starling relationship between CO and LVEDV. Increasing volume initially leads to improved myocardial performance, but volume overload eventually leads to decreased organ perfusion and increased heart strain. CO cardiac output, LVEDV left ventricular end diastolic volume
The current goal of fluid management is to support the cardiovascular system in maintaining cardiac output and thus adequate oxygen-carrying capacity to equal the metabolic demand. Both hypovolemia and hypervolemia decrease tissue perfusion and can result in end organ failure; consequently optimizing volume status does not mean maximizing. Therefore, prior practices of administering excessive levels of fluid to replace preoperative fasting and overestimated insensible losses during the surgical procedure may not be prudent. Rather an individualized fluid regimen, which includes replacing the intravascular and extravascular losses as demanded, should be used.
Measuring and Monitoring Fluid Losses
Accurately measuring the amount of fluid in these compartments is not easily performed; therefore, physical examination, laboratory measurements, and hemodynamic monitoring are utilized to determine a patient’s volume status. Physical examination of hypovolemia include examining the hydration of mucous membranes (dryness), loss of skin turgor, low urine output (<0.5 ml/kg/h), heart rate (tachycardia, >100 beats/min), hypotension (measuring orthostatic blood pressure, if possible), tachypnea, and altered mental state (drowsiness, lethargy). However, under anesthesia often these exam findings are not reliable, as the stress of surgery and many of the medications used for anesthesia alter their relationship with intravascular volume. When the physical exam is unreliable, laboratory measurements such as rising hematocrit, metabolic acidosis, mixed venous oxygen saturation (SvO2), increasing lactate, increasing specific gravity, urinary sodium less than 20 meq/l, urinary osmolality >450 mosm/kg, rising plasma sodium levels, and a BUN-to-creatinine ratio more than 10:1 may be signs of volume depletion.
Invasive monitoring used to determine volume status includes central venous pressure (CVP) monitoring, pulmonary artery catheters (PACs), and arterial lines. CVP is the most widely used invasive technique for measuring intravascular volume in critically ill patients. The CVP is measured by placing a catheter within a central vein at the junction of the superior vena cava and the right atrium. The best time to record CVP is at end expiration, unless the patient is mechanically ventilated in which case true CVP is best measured at the beginning of expiration. The Society of Critical Care Medicine’s Surviving Sepsis protocol recommends a goal of CVP between 8–12 mmHg and 12–15 mmHg for patients on mechanical ventilation. PACs are used to measure both right and left heart pressures by “floating” a balloon-tipped catheter through the right heart to within the proximal branches of the pulmonary artery—preferably the West Lung Zone III. However, multiple studies have shown inconsistencies of PAC measurements in the critically ill. Over the last decade this form of monitoring has fallen from favor with CVP becoming a valid replacement.
Furthermore, arterial line placement can be utilized to determine systolic pressure variability (SPV) within the tracing displayed on monitor (Fig. 7.3). In a mechanically ventilated patient, the baseline pressure is taken during an apneic period. Then the two components of SPV can be measured from the change in peak amplitude with expiration (delta up) and inspiration (delta down). The sum of delta up and delta down equals SVP with a value less than 5 mmHg signifying the absence of hypovolemia. All in all, while CVP, PACs, and arterial lines are useful, they should only be used in conjunction with physical examination and laboratory data to accurately determine volume status and fluid responsiveness.


Fig. 7.3
Determination of systolic pressure variation (SPV)
New literature describes less invasive monitoring of volume status via echocardiography and esophageal Doppler monitoring (EDM) with increasing popularity. Echocardiography when available can be used to visualize the inferior vena cava (IVC) via a subcostal view. A decrease in IVC diameter more than 50 % with respirations correlates with hypovolemia. Furthermore, in the mechanically ventilated patients, an IVC variation of more than 12 % with respirations represents those who would respond to fluid boluses. Esophageal Doppler monitoring (EDM) consists of a small, thin, flexible probe, much like a nasogastric tube, with a Doppler transducer located at the tip, which is placed approximately 35 cm into the esophagus. EDM is used to measure the length traveled by an erythrocyte within the descending aorta during a single cardiac cycle. This correlates to a wave tracing representing flow time and peak velocity. The area under the curve correlates with stroke distance (Fig. 7.4). From this data, the stroke volume can be calculated by multiplying stroke distance and the diameter of the aorta, which is calculated via nomograms based on the patient’s weight and height. Furthermore, cardiac output can be roughly calculated by multiplying the stroke volume by heart rate. These data can be used to determine the appropriate timing and amount of fluid to administer.


Fig. 7.4
Determination of cardiac output by analyzing flow tracing of an esophageal Doppler probe. The area under the curve is calculated (AUC), which shows the stroke distance (the length traveled by an erythrocyte during the cardiac cycle). By multiplying stroke distance by aortic cross-sectional area, the stroke volume is calculated. Cardiac output = stroke volume × heart rate
Fluid Therapy
Fluid resuscitation is a major aspect of managing the care of critically ill patients, whether as a result of burns, trauma, surgery, or from sepsis. There are many different options when it comes to replacing this deficit including crystalloid or colloid solutions, or both. Largely, the difference between crystalloid and colloid is that crystalloids are made from low-molecular-weight salts, while colloids contain larger-molecular-weight proteins or glucose polymers.
Crystalloid solutions include varying concentrations of normal saline (sodium and chloride), lactated ringers (sodium chloride, potassium, calcium, lactate), and dextrose in water (Table 7.2). Normal saline is usually the initial fluid choice for resuscitation. However, excessive amounts of normal saline administered (>3–4 l) can lead to hyperchloremic metabolic acidosis. Lactated ringer’s (LR) solution is slightly hypotonic, providing 100 ml of free water per liter, and the most physiologic solution when a large amount of fluid is required. However, LR is not an ideal solution to use as a Dilutent for administering packed red cells as it contains calcium (antagonizes citrate). Additionally, LR contains potassium, which may not be preferable for patients with kidney disease, and also lactate, which is metabolized to bicarbonate leading to metabolic alkalosis. Dextrose in water is mainly used for replacement of pure water deficits and for hypernatremia but is also useful in preventing ketosis and hypoglycemia during fasting. Dextrose solutions are not used in neuroanesthesia, as hyperglycemia has been found to be detrimental to injured neuronal tissue. Crystalloid solutions, because of their lower molecular weight, lack the ability to maintain the oncotic pressure of the intravascular space and largely (80 %) redistribute throughout the entire extracellular space.
Table 7.2
Composition of commonly used crystalloids
Crystalloid | Tonicity (mosm/l) | Na+ (meq/l) | Cl– (meq/l) | K+ (meq/l) | Ca2+ (meq/l) | Lactate (meq/l) | Glucose (g/l) |
---|---|---|---|---|---|---|---|
Normal saline | 308 (isotonic) | 154 | 154 | ||||
Lactated ringers | 274 (slightly hypotonic) | 130 | 109 | 4 | 3 | 28 | |
5 % Dextrose in water | 253 (hypotonic) | 50 | |||||
D51/2NS | 432 (hypertonic) | 77 | 77 | 50 | |||
3 % NS | 1,026 (hypertonic) | 513 | 513 |
Colloids are organized into naturally occurring albumin and artificial solutions containing starches (dextran, hetastarch) and gelatins. Human albumin is a protein mainly responsible for sustaining intravascular osmotic pressure and should theoretically be the ideal colloid for restoring protein loss from the vasculature. Albumin is prepared by heating it to 60 °C for 10 h, and therefore, an albumin solution does not cause allergic reactions or immunological complications. Furthermore, albumin (5 and 25 % solutions) can add a survival benefit when used to correct hypoalbuminemia in patients with cirrhosis, ascites, and hepatorenal syndrome.
Hydroxyethyl starches are similar to gelatins in that they are artificial, but these are derived from amylopectin which is a glucose molecule found in waxy maize or potatoes. Hetastarch solution (6 % Hespan in isotonic saline) is usually given in a dose of 500–1,000 ml (20 ml/kg). However, it should be noted that hetastarch solutions, by using thromboelastography, were found to cause coagulopathies, specifically depleting von Willebrand factor and factor VIII and prolonging PTT and INR. In 2013, the FDA issued a black box warning for use of Hetastarch in critically ill patients, and in patients with pre-existing renal and severe liver dysfunction (causing excessive bleeding and increased mortality). Dextrose starches (dextran) improve blood flow in the microcirculation by decreasing blood viscosity. However, dextrans have antiplatelet effects (inhibition of aggregation), prolong bleeding time, interfere with blood typing, and cause allergic reactions. Gelatins are polydispersed polypeptides created from degraded bovine collagen, have the potential to cause allergic reactions (including anaphylaxis), and are not available in the United States. Furthermore, Hespan, gelatins, and dextran solutions were shown in multiple studies to cause nephrotoxicity.
There has been a debate through the years as to which solution is superior for resuscitation strategies, crystalloid or colloid. A Cochrane review published in 2011 looked at 65 trials and determined that there was no evidence from randomized control trial’s (RCT) that colloids provided any mortality benefit over crystalloids for patients suffering from trauma, burns, or following surgery. Furthermore, the SAFE trial disproved the belief that larger infusions of crystalloids are required to reach the same resuscitation goals as can be achieved by smaller volumes of colloid. Therefore, because colloids provide no mortality benefit and are considerably more expensive than crystalloid solutions, the value of their continued use in resuscitative guidelines and intensive care management algorithms is not clear.
Preoperative oral hydration
In the 1990s long preoperative fasting strategies were used in patients undergoing elective surgery to avoid emesis and pulmonary aspiration during the induction of general anesthesia. This practice was later questioned, and in 1999 the American Society of Anesthesiologists (ASA) issued new guidelines (see Chap. 2) in which patients were allowed to have clear fluids until 2 h before surgery. Subjectively, the majority of subjects reported to feel more comfortable leading up to their procedure, feeling less thirsty and hungry. All in all, oral rehydration up to 2 h before surgery has shown to increase patient satisfaction without causing any detrimental affects when compared to prolonged fasting in patients.
Strategies for intraoperative fluid replacement
(i)
Traditional method of fluid replacement
Hourly maintenance rate: the 4/2/1 formula:
0–10 kg—4 ml/kg/h
11–20 kg—add 2 ml/kg/h
For each kg above 20 kg—add 1 ml/kg/h for each kg above 20 kg
For example, a 70 kg patient will have an hourly maintenance rate of 110 ml/h (40 + 20 + 50). Therefore, a patient who has been fasting for 10 h has a preexisting fluid deficit of 1,100 ml.
Intraoperative fluid loss: these include evaporative and redistributive (internal redistribution of fluids) fluid losses. These are added to the hourly maintenance rate:
Minor surgery—2 ml/kg/h
Intermediate surgery—4 ml/kg/h
Major surgery—8 ml/kg/h
Replacement of blood loss: intraoperative blood loss can be roughly estimated by measuring the blood in the surgical suction canister (deduct irrigation fluid), fully soaked sponges (10 ml/4 × 4 sponge), and laparotomy pads (100–150 ml). Serial hematocrits can be helpful in estimating blood loss, but are unreliable due to rapid fluid shifts; therefore, serial hematocrits represent more of a ratio of red blood cells to the plasma:
Replace 1 ml of blood loss with 3 ml of crystalloid (half-life of crystalloids = 0.5–1.5 h).
Replace 1 ml of blood loss with 1 ml of colloid (half-life of colloid = 4–6 h).
Replace 1 ml of blood loss with 1 ml of packed red blood cells.
Trigger for blood transfusion is a hemoglobin of 7 mg/dl (10 mg/dl in elderly patients, and in those with significant cardiac/pulmonary disease). However, the decision to transfuse should also take into account the physiologic state of the patient, blood pressure, heart rate, and presence of any acidosis. Approximately, one unit of packed red cells transfused increase the hemoglobin by 1 mg/dl (hematocrit by 3 %).
(ii)
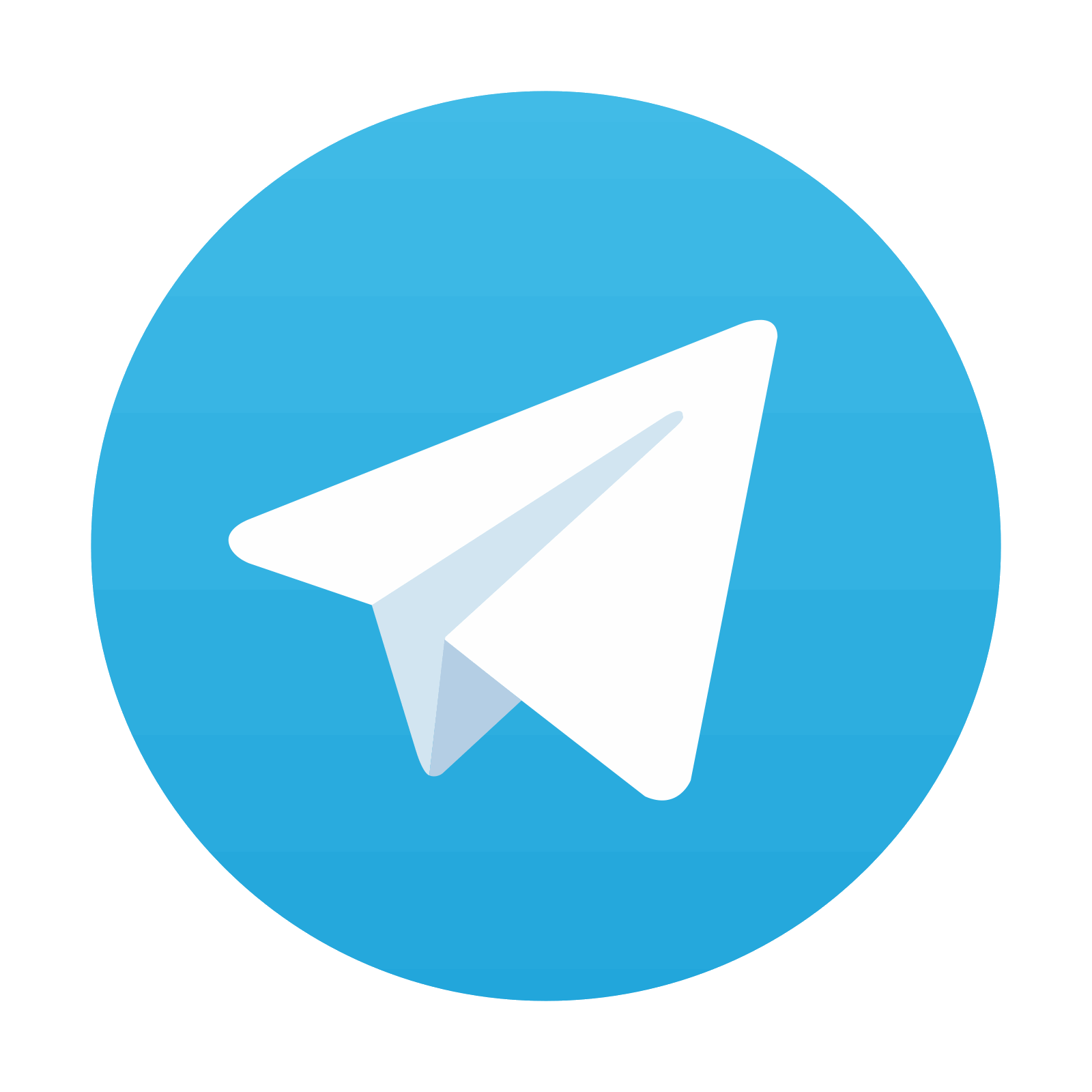
Goal-directed fluid therapy
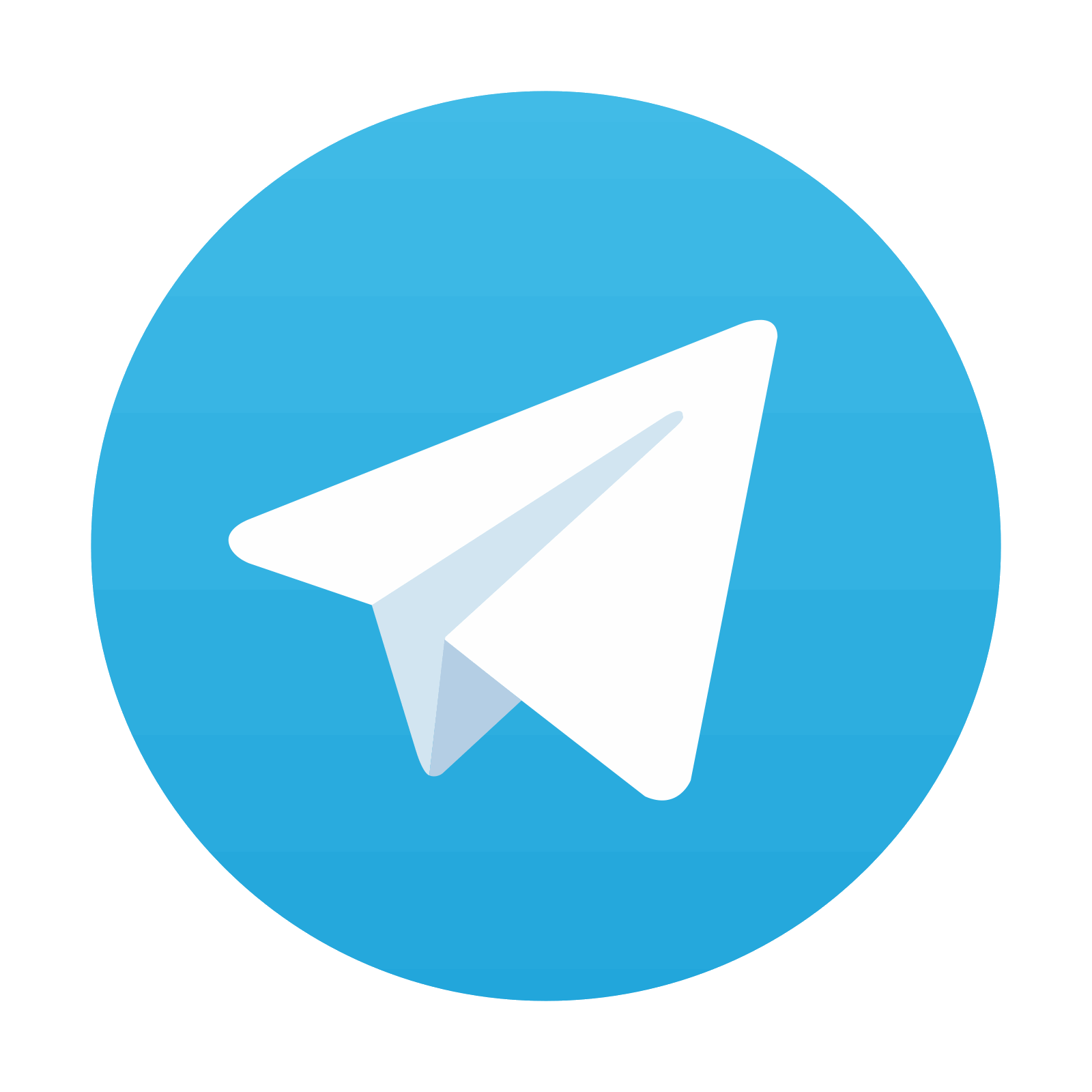
Stay updated, free articles. Join our Telegram channel
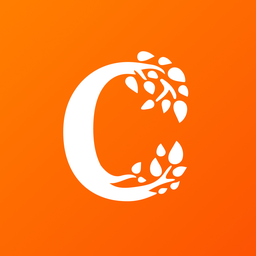
Full access? Get Clinical Tree
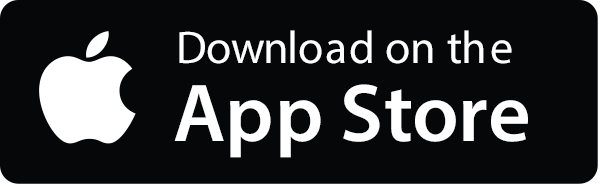
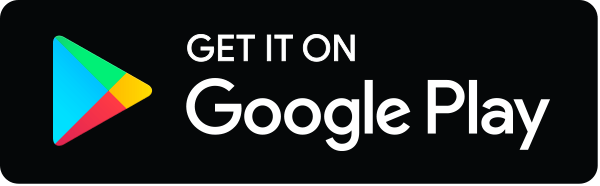
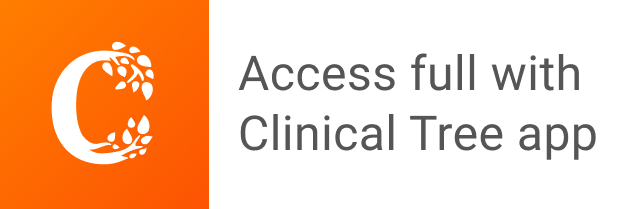