div class=”ChapterContextInformation”>
3. Blood Failure: Pathophysiology and Diagnosis
Keywords
Blood failureShockOxygen debtOxygen deficitMicrocirculationEndotheliumTraumaTrauma-induced coagulopathyHemorrhageTraumatic shockThromboelastographyViscoelastic Hemostatic assayPlatelet dysfunctionLactateHemostasisRemote damage control resuscitationHemostatic dysfunctionDamage control resuscitationIntroduction
Hemorrhage is the leading cause of preventable death from trauma in both civilian and military environments [1, 2]. It is estimated that hemorrhage may account for up to 90% of all potentially salvageable combat-related deaths in the US Military. Traumatic shock is a unique condition combining mechanical tissue injury with hemorrhage so that even organs that are not primarily affected by direct mechanical injury can be primarily and secondarily impacted by hemorrhage wherein these organs can fail in their primary function and in turn further impact other organ systems [3].
A convergence of military conflicts and science over the last 15 years, much of it coming from lessons learned from combat surgery combined with fundamental principles of forgotten physiology, has begun to transform our understanding of traumatic shock and our approach to its treatment. Among these is the recognition that impaired coagulation in the setting of traumatic shock that increases hemorrhage has been identified in 20–30% of trauma victims shortly after injury and when present can increase the incidence of organ failure, intensive care utilization, and even death [4]. This Trauma Induced Coagulopathy (TIC) has resulted in new treatment strategies such as Damage Control Resuscitation (DCR) which rely heavily on transfusion medicine therapies [5–7].
These insights have led to a new and growing concept that blood should be considered an organ system and that like other organ systems, when injured sufficiently it can fail and its failure, can in turn cause injury to other organs [8, 9]. Body organs are composed of distinct populations of cells integrated in a manner allowing them to perform certain critical functions. Blood consist of distinct cell types (red blood cells, white blood cells, platelets) and noncellular components (plasma). However, it is also intimately and inextricably integrated with the vascular endothelium which it constantly bathes and communicates with, each regulating many of the functions of the other. Thus, the blood-endothelial unit can be viewed an as organ system and is unique in that it connects all other organ system.
We will define hemorrhagic blood failure as an emergent state of blood leading to hemostatic dysfunction and a bleeding phenotype resulting from the physiologic and biochemical exhaustion of the blood-endothelium interface caused by a combination of hemorrhage-driven shock and tissue hypoxia, tissue injury, and blood cellular and plasma component loss.

Considering Blood as an organ: Framework for understanding the initiation, evolution, and sustainment of blood failure. (From White et al. [9], with permission of Wolters Kluwer Health, Inc.)
These concepts will assist in understanding the basis for DCR and remote damage control resuscitation (RDCR) including the practice of hemostatic resuscitation. In the setting of traumatic hemorrhage, while there is no substitute for definitive surgical hemostasis, therapy provided prior to this time can greatly impact outcomes, including how rapid hemostasis can be achieved once surgery starts. This pre-surgical resuscitation phase or RDCR and DCR are designed to limit ongoing hemorrhage and to produce or preserve an adequate level of physiologic reserve to deliver a casualty to the hospital that can be salvaged with the follow-on strategy of DCR and Damage Control Surgery (DCS).
The Primary Role of Shock and Oxygen Debt
Victims of severe trauma who develop trauma-induced coagulopathy (TIC) prior to fluid resuscitation or the development of hypothermia show significant evidence of hypoperfusion as a function of elevated base deficits and lactate levels [5, 10, 11]. This is not surprising since severe shock resulting in tissue hypoperfusion manifested by elevated base deficits and lactate levels on admission have been known to be an independent predictor of morbidity and mortality after trauma. Hypoperfusion does not necessarily equate with the severity of injury as reflected in Injury Severity Scores although concomitant severe injury exacerbates the coagulopathy [12–15]. TIC can be exacerbated by plasma dilution with crystalloid resuscitation, hypothermia, and acidosis, which can be iatrogencially produced [5]. Certain traumatic tissue injuries such as those produced by blast and high velocity mechanisms as well as brain injuries may cause and/or exacerbate coagulopathy [16–18]. Many times it is difficult to distinguish their independent contributions to TIC.
Shock is traditionally defined as tissue oxygen delivery below tissue oxygen metabolic needs or demands. While conceptually easy to appreciate, it is important to have a deeper qualitative and quantitative understanding of shock which will impact resuscitative strategies and outcomes as they relate to blood failure. Shock caused by hemorrhage as well as other insults leading to tissue hypoperfusion and hypoxia results in tissues accumulating oxygen debt [19–22].
To date, oxygen debt is the only physiologic indicator that has clearly been linked to both morbidity and mortality in the form of multiple organ failure after shock [19–24]. The degree (depth and length) of oxygen debt have clear consequences as oxygen debt has been linked to the degree of reperfusion injury, inflammation, and acidosis. Oxygen debt can be viewed as a quantitate measure of whole body ischemia. A simple corollary at an individual organ level would be stroke or acute myocardial infarction where the greater the ischemic burden over time, the greater the final tissue damage and function during and after reperfusion.

The biphasic relationship of oxygen delivery (DO2) and oxygen consumption (VO2). As DO2 decreases, VO2 may remain constant due to an increase in the ratio of extracted oxygen (OER) at the tissue level. This is mirrored by a decrease in the venous hemoglobin oxygen saturation (SvO2). However, at some point, OER will not meet VO2 demands of the tissues resulting in a state of DO2-dependent VO2, whereby aerobic VO2 transitions largely to anaerobic VO2. At this point of critical DO2, an oxygen deficit occurs and an oxygen debt begins to accumulate reflected by an increase in byproducts of anaerobiosis such as lactate. While this biphasic relationship exists for the body as a whole, it also exists for each individual organ system. (From Ward [33], with permission of John Wiley and Sons)

Relationship between oxygen deficit and oxygen debt. Oxygen deficit is the difference between VO2 (indicated by the dashed line) and VO2 measured at a given time point (indicated by numbered arrows) during the shock period. The magnitude of the deficit is indicated by the length of the arrow. Oxygen debt is the accumulated sum of all oxygen deficits incurred and is represented by the area under the curve. It should be noted that halting the accumulation of the deficit does not repay back the accumulated debt. (From Barbee et al. [19], with permission of Wolters Kluwer Health, Inc.)

Setting the stage for reperfusion injury. Hemorrhage-induced ischemia leads to electron build up and leak from the mitochondrial membrane (Panel a). The reintroduction of oxygen into previously ischemic tissue produces a host of reactive oxygen species (ROS) such as superoxide and hydroxyl radical and other compounds such as peroxynitrite which can induce signaling and cause oxidative damage to tissues and their cellular machinery (Panel b). (Adapted with permission from Best [120]; Weidinger and Kozlov [27])
Adrenergic activation and catecholamine release induced by pain, neurovascular compensation, and tissue injury exacerbates tissue hypoxia secondary to vasoconstriction and endothelial injury during hemorrhage thus increasing oxygen debt [29]. Tissue injury with activation of pain and adrenergic pathways increase oxygen debt in the setting of hemorrhage over hemorrhage alone [30]. Adrenergic activation and catecholamine release also stimulate lactate production by activation of the Na+-K+-ATPase so that blood lactate levels are elevated and may be used as a supplementary tissue fuel source for vital organs [31].
Since it is well-known that reperfusion injury drives subsequent inflammatory and immune dysfunction , it is important to underscore the strong relationship that exists between the degree/depth of oxygen debt and ensuing inflammation during the subsequent reperfusion injury. However, instead of being limited to a single organ, the insult affects multiple organs directly and indirectly via the reperfusion injury cascade [22, 32]. This will include the blood via damage to the endothelium as well as circulating coagulation proteins such as fibrinogen [33].
A common but very overlooked and misunderstood physiologic principle relating to oxygen debt is the need for timely repayment of a critical portion of oxygen debt [19–21, 24]. Similar to sleep and other physiologic debts, it is not possible to incur a significant oxygen debt with no consequences if it is not repaid. Halting the oxygen deficit by simply returning to a DO2- independent VO2 state, while essential is not sufficient in and of itself to achieve homeostasis (Fig. 3.3). In addition to meeting the basal metabolic demands of the body to restore aerobic metabolism and halt additional oxygen deficit accumulation, additional consumption is required to replenish critical cellular energetic processes including the phosphogen and glycogen-lactic acid system which can be significantly depleted during shock [34]. The ability to repay these systems in a timely manner decreases significantly with increasing levels of debt and time.

Relationship between various oxygen debt repayment profiles and the likelihood of significant organ damage and/or death. Oxygen debt is represented by the area of the basal VO2 dashed line; oxygen debt repayment is the area above the basal VO2 dashed line. Resuscitation is marked by the dotted vertical line. The more rapid that critical portions of debt can be repaid (a), the better chance of survival and survival without organ failure. Delayed repayment (b and c) can result in varying levels of organ failure and ultimately death. The exact proportion of debt and the kinetics of repayment to avoid death and organ failure are not clearly known and there are challenges due to lack of technology in knowing both how much debt is accumulated and how much is repaid. (From Barbee et al. [19], with permission of Wolters Kluwer Health, Inc.)
The principles of oxygen debt and its contribution to blood failure can serve as a basis for judging the limitations of various resuscitation strategies such as permissive hypotension in developing new resuscitation solutions or protocols. Their effects on limiting oxygen debt and in promoting repayment of critical portions to prevent or reverse blood failure are critical to consider. An understanding of oxygen debt also invites the opportunity to consider modulating oxygen consumption such as decreasing consumption through reducing metabolism as a means for reducing oxygen debt accumulation or in repaying debt more quickly.
The Microcirculation and Endothelium
The linkage between oxygen debt and traditional organ failure (hepatic, renal, lung, etc.) has been long recognized. It is therefore intuitive that the endothelial portion of the blood-endothelial unit is also affected by oxygen debt. The microcirculation with its endothelial lining is estimated to cover an area of up to 7000 m2 and thus represents what might represent the body’s largest organ system [36, 37]. The individual microcirculatory unit composed of the arteriole, capillary bed, and postcapillary venule is designed to ensure the delivery of oxygen and other nutrients to tissues in excess of their needs as well as to remove products of metabolism. With an estimated 1013 endothelial cells in an adult, the endothelium is constantly exposed to blood [36]. It is, therefore, logical that it would be prone to significant cellular injury resulting in dysfunction from trauma, hemorrhage, hypoperfusion, reperfusion injury, and inflammation [38, 39]. Complicating our understanding is the fact that there is no universal phenotype for the endothelium and that this phenotypic heterogeneity and the role it plays is likely to simply be a core property as it is with the parenchymal cells of other organ systems [38, 39]. For example, arteriole endothelium plays a major role in regulating vascular tone through signal transduction via shear stress to the vascular smooth muscle, while postcapillary venule endothelium is involved in leukocyte trafficking in response to injury [40]. Thus, injury to and dysfunction or failure of the endothelium can be termed “endotheliopathy” and thought of similarly to how we view injury to and impaired function of other organs (cardiomyopapthy, etc.). This endotheliopathy can be characterized by three main components: (1) paracellular permeability, (2) dysfunctional hemostasis, and (3) inflammation [41–43].
The endothelium regulates the integrity of the blood-organ barrier and clot formation including both anticoagulant and procoagulant functions. Endotheliopathy secondary to hypoxia results in endothelial cell surface activation by inflammatory mediators, in addition to adhesion of platelets, red blood cells, and leukocytes to activated endothelial cells. Endothelial cell activation also increases the production of coagulation pathway intermediates [9].
Under normal physiologic conditions , the endothelial cell surface maintains blood fluidity and regulates flow by multiple anticoagulant mechanisms. The surface-linked protein thrombomodulin binds thrombin converting it from a potent procoagulant to an anticoagulant by increasing its affinity for protein C above that of fibrinogen [44, 45]. Activation of protein C can further reduce Factor V and VII levels. Once activated, protein C is also capable of interacting with endothelial cells to activate cell survival responses and maintain the endothelial barrier [46]. Synthesis of local prostacyclin (PGI2) and ADP metabolism to adenine nucleotides also inhibit platelet activation, adhesion, and aggregation. Vascular patency is impacted and regulated by the endothelium synthesizing nitric oxide which causes vasodilation to keep the microcirculation open. Nitric oxide also inhibits platelet function and tPA, which activates plasminogen to plasmin, the primary proteolytic enzyme of fibrin [41, 46]. As a result, nitric oxide formation secondary to hypoperfusion has competing effects on hemostasis.
Paracellular permeability leading to organ edema and failure is caused by hypoxia-induced breakdown of endothelial cell-cell tight and adherent junctions that regulate the endothelial blood-organ barrier in various tissues. While tissue specific, these barriers are, in general, maintained by structural components (i.e., adherens junctions), cellular components (i.e., smooth muscle cells and pericytes), and extracellular matrix proteins, all working to maintain the endothelium barrier [9]. This is important as the stress of hypoperfusion secondary to traumatic hemorrhage leads to antagonistic signaling of angioproteins 1 and 2 signal via the Tie-2 receptor to tighten (Ang-1) or loosen (Ang-2) the barrier that separates the strong procoagulant subendothelial tissues from blood [47]. An additional layer of protection is afforded by a thick surface matrix made up of membrane-bound glycoproteins and proteoglycans having heparin-like activity called the glycocalyx . The glycocalyx is a protective border on endothelial cells that regulates endothelial cell permeability and shear stress, meant to limit the interactions of the endothelium with circulating blood cells which in turn inhibits local thrombin activity [48]. However, with traumatic injury and severe shock, the injured endothelium initially promotes coagulation due to barrier disruption when activated by releasing Ang-2, tissue factor, von Willebrand factor, platelet activating factor, and PAI-I [48]. Thus, the balance of the state of quiescent anticoagulant or activate procoagulant phenotypes of the state of the endothelium is dependent on the degree of local and system injury.

Effect of hemorrhagic shock on the endothelial glycocalyx. Absence of the endothelial glycocalyx following hemorrhagic shock in a rat (left). Endothelial glycocalyx of experimental sham rat (right). (From Kozar and Pati [51], fig. 1, with permission of Wolters Kluwer Health, Inc.)
Glycocalyx shedding enables direct interactions between inflammatory blood cells and their mediators with the direct surface of the endothelium. This exposure is also capable of activating platelets as evidenced by increases in the platelet-derived inflammatory mediator soluble CD40 ligand that is also associated with sympathoadrenal and immune system activation and increased mortality [54]. Activated neutrophils migrate to the endothelial surface and contribute to oxidative damage of the surface through the release of neutrophil extracellular traps [55]. Endothelial cell surface oxidation may also promote direct red cell adhesion inducing local thrombosis and further microcirculatory hypoperfusion [56]. Lastly, oxidative stress from neutrophils is believed to directly impact circulating coagulation factors. For example, oxidation of a single key methionine in the Aα-C domain of fibrinogen to methionine sulfoxide by hypochlorous acid, produced by activated neutrophils can disrupt fibrin polymerization [57, 58]. Elucidation of the complex but integrated components of the blood-endothelial unit continue to argue for the concept and critical understanding of blood failure.

Endotheliopathy of traumatic shock (see text for details). (With permission, Johansson et al. [121])
Blood Failure and Coagulopathy
Clinical observation strongly supports that coagulopathy as a manifestation of hemorrhagic blood failure is a serious evolving condition composed of many local and systemic responses to injury and shock. Primary among these responses in the release of tPA to activate fibrinolysis [59]. In addition, soluble thrombomodulin and activated protein C levels are increased. However, activated protein C levels are not sufficient in and of itself to anticoagulate normal plasma [60]. Platelets are also capable of activating sufficient FV to support hemostasis and overcome supraphysiologic levels of activated protein C [61]. While the procoagulant activity of thrombin generation is generally accepted to be elevated in trauma patients (even in the setting of coagulopathy) above those of healthy controls, the blood of trauma patients having prolonged prothrombin time/international normalized ratio (PT/INR) may not be truly reflective of reduced thrombin formation [62–64]. Abnormalities in plasma-based clotting assays like PT/INR may instead reflect complex interactions of fibrinogen depletion, fibrin degradation product interference with fibrin mesh formation, dysregulated thrombin generation, and excess plasmin activity manifested most obviously in fibrinolysis [9].
Perhaps most devastating in the evolution of hemorrhagic blood failure is increased proteolytic activity within blood. Multiple circulating proteolytic enzymes are increased after trauma including neutrophil elastase and plasmin [65]. Plasmin is a serine protease having proteolytic activity against a wide range of coagulation proteins, membrane proteins, and integrins. Plasmin proteolysis can activate FV, FVIII, and FXIIIa and can activate FXII, thus linking its activation to complement, inflammation, and immunity by direct generation of bradykinin from high molecular weight kininogen [66–68]. However, the most direct effect of plasmin on coagulation is its activity against both fibrinogen and fibrin, which contributes to rapid fibrinogen consumption and fibrinolysis after traumatic shock [69, 70]. The degree of fibrinolysis is positively associated with mortality and it appears to be proportional to the degree of shock [71].
Overall, severe trauma accompanied by shock appears to increase both thrombin generation and plasmin activation. While there may be some benefit to physiologic fibrinolysis after trauma, both an early increase in fibrinolysis and a later resistance to fibrinolysis are associated with increases mortality during traumatic shock [72]. Thus, while some degree of fibrinolysis may support vascular patency and tissue oxygen delivery during low flow states, it also exacerbates blood loss prior to surgical hemostasis. Studies such as the CRASH-2 trial demonstrate a mortality benefit in the setting of hemorrhage when tranexamic acid (an antifibrinolytic) is administered early after hemorrhage strongly suggesting that shock-induced blood proteolysis is an important component of coagulopathy and driver of blood failure [73]. Underscoring the integrated nature of the blood and endothelium as an organ system is that inhibition of plasmin activation with tranexamic acid in traumatic shock also appears to improve endothelial barrier function, reducing tissue edema and injury due in part to decreased bradykinin generation [74].
Another critical contributor to traumatic hemorrhage- induced blood failure is platelet dysfunction. Circulating platelets act to initiate clot formation at wounds by adhesion and aggregation during primary hemostasis. In addition, they provide a local environment that supports thrombin generation where they forcefully contract fibrin to stabilize evolving clots. When measured by impedance aggregation and viscoelastic methods, hemorrhage- induced platelet dysfunction has been strongly linked to mortality [75, 76]. The discreet mechanisms by which platelet dysfunction occurs is unclear given that the surface of dysfunctional platelets also seem to be paradoxically activated [77]. Since platelet-induced clot contraction is critical to clot strength after injury and hemorrhage, it explains the variability in clotting strength profiles encountered in the early time periods after trauma and resuscitation [78].

Schematic of key linkages between oxygen debt, cellular dysfunction, and coagulopathy during hemorrhagic-induced blood failure. (From White et al. [9], with permission of Wolters Kluwer Health, Inc.)
Diagnosis and Monitoring of Blood Failure
Traumatic blood failure is defined as a cascading series of events within the blood compartment induced by injury and blood loss that culminate in the loss of the vital functions of the blood. Hemostasis is one vital blood function that can become dysfunctional contributing to increased mortality after severe injury. As explained earlier, the pathophysiology of altered hemostasis is complex and linked to a series of events initiated by traumatic shock.
It should be emphasized that the major factor initiating blood failure and its severity is the magnitude of traumatic shock. Rapid halting of oxygen-deficit accumulation and repayment of oxygen debt are essential in limiting the degree of blood failure. The ability to monitor for each aspect of blood failure would enhance the rapid application of therapies to prevent or treat it. Unfortunately, there are few readily available monitoring methods that have been demonstrated to be effective in accurately and rapidly detecting shock, hemostatic, immune and endothelial dysfunction.
Perhaps the most well-known tool that is beginning to move beyond the trauma center into the field is the use of point of care (POC) or near POC monitoring of whole blood lactate. As discussed earlier, lactate is capable of tracking the accumulation and degree of oxygen debt during injury, but it requires serial measurements [22, 79–81]. The quantitative and temporal ranges and limits of lactate to predict blood failure in humans have not been well defined. In addition, given the biphasic nature of the relationship between oxygen delivery and consumption, clearance of lactate does not ensure repayment of oxygen debt [19]. Experimentally, it appears that the faster lactate clearance is, the better chance there is of repaying debt or at least of critical portion of debt that improves survival [24, 82]. Thus, trajectory of clearance is important. Elevations of lactate should prompt aggressive efforts to halt hemorrhage and to provide a level or resuscitation that limits further lactate accumulation and begins to clear lactate. Use of permissive hypotension in the RDCR or DCR setting pose challenges to this strategy and must be balanced with the need to limit ongoing hemorrhage as well as the availability of blood products to use in resuscitation.
Other potential useful tools include tissue hemoglobin oxygen saturation (StO2) and compensatory reserve index (CRI) monitoring . Based on the relationship between oxygen delivery, oxygen consumption, and oxygen extraction, the use of StO2 monitoring is attractive. Spectroscopy techniques including near infrared spectroscopy (NIRS) and resonance Raman spectroscopy (RRS) attempt to exploit the fact that the majority of blood volume within a tissue is 70–80% venous [83]. The ability to monitor aggregate StO2 of a tissue is thus representative of the postextraction portion of the tissue compartment similar to monitoring of central or mixed venous oxygen saturation (Fig. 3.2). Thus, the potential exist for StO2 to detect reductions in tissue oxygenation prior to reaching critical DO2 as well as to ensure that during resuscitation that oxygen extraction ratios is increased into normal ranges helping to ensure that oxygen debt is being rapidly repaid as lactate levels fall [33, 84]. Certain caveats to the use of this strategy exist including choosing a target tissue to monitor that is sensitive to reductions in blood flow and responsive to resuscitation. For NIRS, the use of skeletal muscle beds such as the deltoid or thenar eminence has been used. However, when used in this manner, NIRS will also capture myoglobin oxygenation spectra which cannot be differentiated from hemoglobin. This is important since the p50 of hemoglobin is 5 mmHg versus 26 mmHg for hemoglobin [84]. This fact may prevent NIRS from acting as a sensitive early warning monitoring modality. Its use in trauma to guide treatment has been met with mixed results with wide variability in baseline readings [85, 86]. While RRS using the buccal mucosa appears to overcome these issues, its use is still experimental and has only been tested in preclinical models [87]. If NIRS is used, attempts to normalized StO2 to values above 70% should be made with concomitant tracking of lactate levels. This same strategy should be utilized if monitoring of central venous hemoglobin oxygen saturation (ScvO2) is available in the in-hospital setting. Experimental models indicate that changes in clot strength during hemorrhage are impacted by changes in ScvO2 changes indicating, again, the sensitivity of hemostasis to tissue hypoxia [88].
CRI is a newly developed technique utilizing artificial intelligence to identify and track changes in the photoplethysmograph (PPG) of a pulse oximeter to detect vascular changes associated with cardiovascular decompensation due to central hypovolemia [89, 90]. Preliminary clinical data suggest CRI may be of value in identifying patients who are actively decompensating before changes in traditional vital signs such as systolic blood pressure occur [89]. In one study, abnormal CRI values were found to equal the ability to identify hemorrhage and correlated well with lactate levels themselves [91]. While promising, the ability of CRI to robustly detect and track the accumulation of lactate and its clearance require further study.
In summary, serial whole blood lactate and surrogates such as base deficit are currently the most rapid and efficient means to estimate and monitor the degree of oxygen debt and its resolution associated with traumatic shock and its resuscitation and should be used in conjunction with the coagulation-based laboratory methods described below. Laboratory methods have been used to identify and characterize these components of blood failure with variable success. In this section, we review the use of laboratory methods used to identify blood failure with an emphasis on identification of coagulopathy after injury.
Plasma-Based Laboratory Methods
The prothrombin time (PT) assay is a widely used and standard laboratory coagulation assay measuring the extrinsic coagulation pathway activation state. The test is primarily used to manage anticoagulation therapy using vitamin K antagonists. The PT has been used to identify the presence of a distinct “acute coagulopathy of trauma” present in approximately 25% of severely injured trauma patients [4]. A prothrombin time test (PT) of >18 seconds was used as the definition of coagulopathy and was associated with an approximately four- to sixfold increased mortality [4]. Since these initial reports, PT and the PT-based standardized International Normalized Ratio (INR) have continued to be used as a primary laboratory method for identification of coagulopathy. A large multicenter study of PT ratio found that any increase beyond 1.2 represented an increased mortality for trauma patients, increasing from 7% to 22% [92]. However, increased INR may overestimate the presence of coagulopathy in stable trauma and surgical patients [93]. Further increasing the INR cutoff to >1.5 found increased specificity for both hemorrhagic and thrombotic complications in trauma patients [94].
The activated partial thromboplastin time (aPTT) is another standard plasma-based coagulation assay that measures the intrinsic pathway of clot formation. It was developed and is most widely used to manage anticoagulation therapy using unfractionated heparin. Prolongation of aPTT is predictive of mortality in trauma patients where it was found to be less sensitive but more specific [4]. While the aPTT is a part of most standard coagulation panels measured in trauma patients, its use for traumatic coagulopathy and blood failure has been limited. The PT/INR and aPTT are often measured together and are useful as screening tools when one or both are prolonged in trauma patients. However, since procoagulant activity is typically increased in the early stages of traumatic coagulopathy and blood failure, their usefulness to guide specific therapies are limited [62, 63]. This limitation likely stems from the fact that multiple coagulation abnormalities ranging from decreased activity of specific coagulation factors such as Factor V and VIII, endogenous heparinization, and loss of fibrinogen are present in trauma patients and can induce prolongation of one or both of these assays [52, 95, 96]. Thus, they are most useful as screening tools capable of identifying the risk of coagulopathy and should be used to initiate more specific laboratory investigation.
Of particular importance to the detection and treatment of blood failure is the concentration of the hemostatic protein fibrinogen which is negatively associated with mortality after trauma [96]. A popular method for measuring fibrinogen concentration in plasma is based upon the method of Von Clauss, where functional fibrinogen concentration in plasma is measured by its time to fibrin formation after activation by an excess of thrombin after sample dilution [97]. Fibrinogen is rapidly lost from the blood during hemorrhage requiring blood component transfusion, being the first coagulation protein to reach critically low functional levels in experimental models and during surgery with significant blood loss [98]. Trauma patients with decreased fibrinogen concentrations in the emergency department require more blood product transfusions and are more likely to die [96]. Standard cutoff values for fibrinogen replacement have increased steadily as more evidence of its importance has accumulated. Historical cutoff values of 100 mg/dl have increased to a recommended maintenance of 150–200 mg/dl by European guidelines [99]. A more recent large multicenter study of fibrinogen concentration in trauma patients found that mortality doubled when fibrinogen was less than 228 mg/dl, suggesting that replacement thresholds are likely to increase even further [100]. Despite strong associations with clinical outcomes in trauma, fibrinogen concentration is not measured directly in many trauma centers. However, clinically relevant hypofibrinogenemia is common and shows a strong correlation with other standard laboratory parameters such as hemoglobin concentration and base excess, which may indirectly suggest the need for early fibrinogen replacement [101].
Another important contributor to coagulopathy after trauma is increased proteolytic activation in the blood by generation of plasmin from plasminogen. Plasmin is a fairly nonspecific protease that has particular affinity for enzymatic degradation of fibrin. Plasmin’s degradation of fibrin increases the fibrin-specific cleave product D-dimer in blood. D-dimer is commonly used clinically as a screening tool to identify venous thromboembolism in emergency department patients [102]. There is ample evidence supporting significant elevations of D-dimer in blood during blood failure with coagulopathy [71, 103, 104]. However, D-dimer tends to be elevated in most trauma patients, and thresholds for sensitive detection of coagulopathy and blood failure have not yet been reported.
Whole-Blood Laboratory Methods
Common plasma-based laboratory methods used for detection of coagulopathy after trauma cannot consider the cellular contributions to blood failure. This is important because cellular dysfunction, especially of blood platelets, is strongly associated with mortality after trauma [77]. Kutcher et al. used multiple electrode aggregometry to find that decreased platelet aggregation responses to any agonist in emergency department trauma patients was associated with a tenfold mortality increase [75]. Platelet contraction is also a primary contributor to changes in clot formation in emergency department trauma patients [18]. In addition, changes in hematocrit, mostly determined by red blood cell mass, can also directly affect clot formation and hemostasis [105, 106].

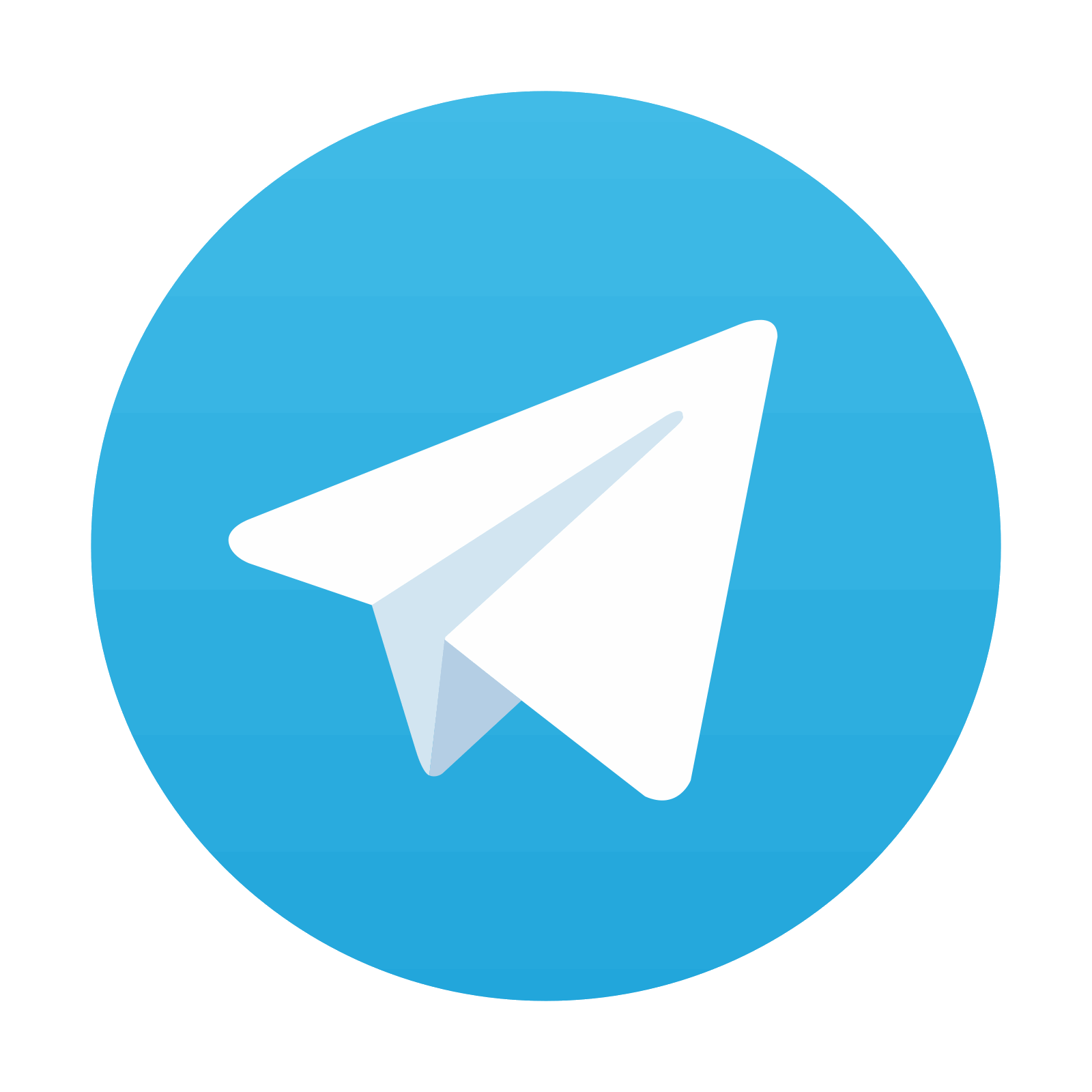
Stay updated, free articles. Join our Telegram channel
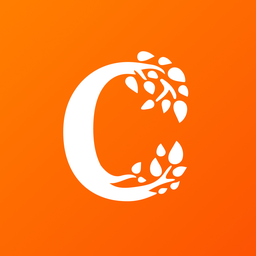
Full access? Get Clinical Tree
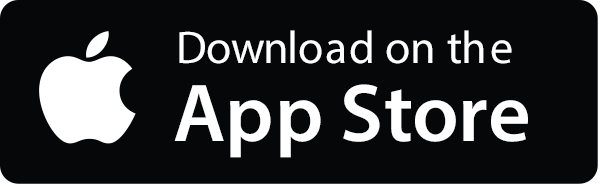
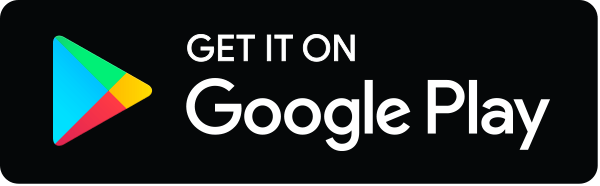