FIGURE 1 Infrared laser stimulation (LS) of heat-sensitive nociceptive afferents of the skin is currently the most commonly used method to elicit nociceptive ERPs in humans. The high energy density of the laser beam allows the generation of very steep heating ramps, producing a highly synchronous afferent volley well suited to record time-locked responses such as ERPs. Contact heat stimulation (CHS) using a thermode applied against the skin has been proposed as a alternative technique to elicit ERPs related to the activation of heat (or cold) sensitive free nerve endings. However, as compared to LS, the heating ramps generated by CHS are not as steep and, hence, the afferent volley is not as synchronous. Punctate mechanical stimulation (PMS) can be used to specifically explore the responses elicited by mechanical nociceptive stimulation. However, these stimuli unavoidably also activate low-threshold mechanoreceptors of the lemniscal pathway. Finally, intra-epidermal electrical stimulation (IES) can be used to generate a very focal electric current selectively activating free nerve endings of the epidermis without concurrently activating more deeply-located non-nociceptive mechanoreceptors. However, the stimulus will be selective for nociceptive afferents only if low intensities are used.
FIGURE 2 Skin temperature profile following the delivery of a short-lasting (50 ms) pulse of radiant heat generated by an infrared laser stimulator. The high energy density of the laser beam allows the generation of a very steep heating ramp, quasi-simultaneously activating heat-sensitive C- and Aδ-fiber afferents. The upper inset shows six consecutive images obtained using an infrared camera. The color scale represents skin temperature from black (baseline skin temperature) to purple (maximum target skin temperature).
Another alternative method to elicit ERPs or magnetic fields related to the selective activation of nociceptors is intra-epidermal electrical stimulation (IES) [43,49,66]. The technique relies on the fact that a dense network of nociceptive free nerve endings is located in the epidermis, while non-nociceptive mechanoreceptors are mainly located more deeply in the dermis. IES could circumvent some limitations of laser stimulation, such as skin overheating and lesion due to stimulus repetition, and delay or relative desynchronization of the nociceptive afferent volley due to transduction of thermal energy into a neural impulse [62]. However, IES suffers from its own limitations, in particular, the need to use low stimulation intensities to guarantee its selectivity for nociceptors. Indeed, it has been shown that if IES is delivered using a strong intensity (e.g. an intensity corresponding to the pain threshold), the stimulus is not selective for nociceptors because it also activates more deeply located low-threshold mechanoreceptors [76]. The important drawback is that, at such low intensities, a single electrical stimulus elicits a very weak sensation and the signal-to-noise ratio of the elicited ERPs is low, possibly because of the very small number of recruited afferents [53]. To circumvent the lack of spatial summation, some authors have proposed to deliver short trains of stimuli (e.g. three pulses delivered at a 5-ms inter-stimulus interval) [44,49] or to use multiple electrodes with the aim of increasing the strength of the nociceptive afferent volley through temporal summation. However, repetition of the electrical pulse could activate some additional (non-nociceptive) fibers brought to a subthreshold potential by the preceding pulses.
Finally, it was recently suggested that mechanical pinprick stimuli delivered using a thin (e.g. 0.25 mm diameter) flat-tip probe could be used to elicit ERPs related to the activation of mechano-sensitive nociceptors [38], although such stimuli inevitably also activate non-nociceptive low threshold mechanoreceptors.
Here, we will focus exclusively on the human electrophysiological brain responses that can be elicited using laser stimulation. In the vast majority of studies, the thermal stimuli used to elicit ERPs or magnetic fields activate Aδ and C nociceptors concomitantly, thus eliciting both a sensation of first pain and a sensation of second pain [55,73,82,101]. Indeed, only a few studies have focused on the event-related brain responses elicited by stimuli activating C nociceptors selectively [80]. Myelinated Aδ fibers and unmyelinated C fibers have very different conduction velocities [73,80,101]. Therefore, if the thermal stimulus activates both classes of nociceptors quasi-simultaneously, it may be expected to generate two distinct nociceptive afferent volleys reaching their respective cortical targets at very different latencies, especially if the stimulus is applied to the distal part of a limb. For example, if a laser stimulus is applied to the dorsum of the hand, the time required to reach the cortex may be expected to be lower than 200 ms for the nociceptive afferent volley conveyed by Aδ fibers and greater than 600 ms for the nociceptive afferent volley conveyed by C fibers. Hence, because electrophysiological techniques sample brain activity with a temporal resolution lying in the range of milliseconds, it is clearly possible to determine whether the latencies of the elicited responses are compatible with the conduction velocity of Aδ fibers (e.g. latency < 650 ms when stimulating the hand dorsum) or with the conduction velocity of C fibers (e.g. latency > 650 ms when stimulating the hand dorsum) [13]. However, laser stimuli concomitantly activating Aδ and C fibers elicit ERPs whose latencies are compatible with the conduction velocity of Aδ fibers, but do not elicit ERPs whose latencies are compatible with the conduction velocity of C fibers [28]. These responses consist of a large negative-positive complex, maximal at the scalp vertex, whose latency, when stimulating the hand dorsum, is approximately 240 ms (negative wave) and 400 ms (positive wave). In summary, although the stimulus elicits a clear double percept related to the co-activation of Aδ and C nociceptors, the electrophysiological correlates of the cortical processing of the thermal stimulus, as reflected by LEPs, only include brain responses compatible with the conduction velocity of Aδ fibers.
Given that ERPs and magnetic fields reflect only brain responses that are triggered by a very phasic sensory afferent volley, it is generally accepted that LEPs reflect only the activity triggered by nociceptors exhibiting very phasic responses to thermal stimulation. For example, Treede et al. [101] showed that mechano- and heat-sensitive Aδ-fiber nociceptors (AMH) can be subdivided into two relatively distinct populations: type I AMH displaying a tonic response to heat consisting in a slow increase of activity peaking only several seconds after stimulus onset, and type II AMH displaying a very phasic response to heat consisting in a strong but very transient response almost synchronous with stimulus onset. For this reason, it is often considered that LEPs and laser-evoked magnetic fields mainly reflect the activation of type II AMH nociceptors.
LASER-EVOKED BRAIN POTENTIALS (LEPS)
Because EEG is a widely available and non-invasive technique, the majority of studies have relied on this technique to study the electrical brain activity elicited by the thermal activation of nociceptors using lasers.
LEPs Elicited by the Co-Activation of Aδ and C Nociceptors
Brief thermal stimuli applied to the skin elicit a large negative-positive potential referred to as the N2 and P2 waves or N2-P2 complex [10] (Fig. 3). Given their large amplitude, the N2 and P2 waves are often visible in single trials, and can be identified and characterized reliably using only a few repeated stimuli (typically, 20-30 stimuli) [16]. The scalp topographies of both the N2 and P2 waves are maximal at the vertex, and are largely independent of the location of the stimulus [29]. The N2 and P2 waves are preceded by an earlier negative deflection, often referred to as the N1 wave [36,99,100,102]. At the scalp vertex, the N1 wave often appears embedded in the ascending shoulder of the N2 wave, and is thus difficult to isolate. The scalp topography of the N1 wave is clearly lateralized, appearing maximal over central and temporal regions contralateral to the stimulated side. Several studies have suggested that the scalp topography of the N1 wave may be further dependent on the stimulation site [102,112]. Indeed, they described a contralateral central or temporal topography when stimulating the hand, and a more medial scalp topography when stimulating the foot, thus suggesting a somatotopic organization of the neuronal populations contributing to the N1 wave. However, it should be noted that several studies examining LEPs elicited by the stimulation of different body districts have failed to identify such a somatotopic shift of the N1 wave scalp topography [94,96,104].
FIGURE 3 Nociceptive event-related potentials elicited by laser stimulation (Nd:YAP), contact heat and cold stimulation, intra-epidermal electrical stimulation and punctate mechanical stimulation. Group-level average waveforms are displayed of the signals recorded at the scalp vertex (electrode Cz) and/or the contralateral temporal region (electrode Tc reference to electrode Fz) of the hand of human volunteers. Note that all waveforms are characterized by a large negative-positive response maximal at the scalp vertex, preceded by a smaller and lateralized negative peak, here visualized at the contralateral temporal electrode (black arrows). Also note the important differences in the latency of the responses elicited by the different types of stimuli. These differences can be explained by differences in transduction times. The laser EPs were obtained using an Nd:YAP laser, which penetrates deep in the skin. For this reason, the responses elicited by stimulation of the hand palm and hand dorsum are highly similar [42]. Contact heat ERPs [42] and cold ERPs [4] elicited by a thermode applied against the skin have a greater latency, explained by the longer time required for the relatively slow heating or cooling ramps to reach activation threshold. In contrast, the ERPs elicited by intra-epidermal electrical stimulation [62] have a shorter latency, explained by the fact that direct electrical stimulation bypasses transduction. Finally, the ERPs elicited by punctate mechanical stimulation [38] also exhibit relatively short response latencies, possibly explained by differences in mechanical and thermal transduction times and/or the concomitant activation of rapidly-conducting non-nociceptive Aβ-fiber mechanoreceptors.
The latency of the N1, N2 and P2 waves of LEPs is largely dependent on the peripheral conductance distance, and compatible with the conduction velocity of Aδ fibers, but incompatible with the conduction velocity of C fibers. Several studies have attempted to estimate the conduction velocity of the spino-thalamic tracts conveying the afferent volley leading to LEPs. For example, by comparing the latencies of LEPs elicited by stimuli applied to the skin overlying vertebral spinous processes at various levels (to minimize peripheral conduction distance), Cruccu et al. [17] estimated that the conduction velocity of the spino-thalamic tracts generating the P2 wave was 11.2 m/s. Using the same method, Valeriani et al. [103] proposed that the conduction velocity of the spino-thalamic tracts generating the N1 wave was significantly slower (9 m/s) than the conduction velocity of the spino-thalamic tracts generating the later P2 wave (13 m/s), and concluded that both waves reflect nociceptive input conveyed within distinct spino-thalamic pathways.
A number of studies have applied source analysis methods to laser-evoked brain potentials [29]. Most of these studies have used methods based on the optimization of a fixed spatio-temporal dipole (e.g. [96]). Other studies have used distributed source models, which reconstruct brain electrical activity in each point of a three-dimensional mesh (e.g. [102]). Apart from some exceptions [6,40], most of these studies have suggested that bilateral opercular regions contribute largely to the obtained waveforms. These opercular sources have been hypothesized to reflect neural activity originating from the secondary somatosensory cortex (S2) and, possibly, from the deeper insular cortex [29]. Most studies have identified the contralateral opercular response as the earliest response following laser stimulation, coinciding with the latency of the N1 wave. However, the suggested time courses of opercular activity suggest that they also contribute to the later N2 and P2 waves. In addition to bilateral opercular sources, source-analysis studies have repeatedly proposed that LEPs also reflect activity originating from the anterior cingulate cortex, in particular, the midportion corresponding to Brodman’s area 24 [29]. In most cases, the latency of this additional source of activity suggests that it contributes mostly to the later N2 and P2 waves. Whether or not the primary somatosensory cortex contributes to the laser-evoked potential waveform remains a matter of debate. Indeed, most source-analysis studies have shown that the bulk of the LEPs can be satisfactorily explained without assigning sources to the contralateral primary somatosensory cortex. However, recent studies have provided evidence that the contralateral primary somatosensory cortex does contribute significantly to the early N1 wave [102]. Furthermore, it was suggested that the contralateral primary somatosensory cortex may also contribute to the ascending portion of the P2 wave [37].
LEPs Elicited by the Selective Activation of C Nociceptors
A number of studies have examined the laser-evoked brain potentials elicited by the selective activation of C nociceptors, using various methods to avoid the concomitant activation of Aδ nociceptors, or to block the transmission of Aδ fiber input at peripheral level [80].
A first method takes advantage of the fact that unmyelinated C fibers are more resistant to focal nerve compression than myelinated nerve fibers, including small-diameter Aδ fibers [9]. Using this differential property, several studies have applied pressure to the superficial branch of the radial nerve to induce a selective blockade of myelinated nerve fibers. These studies have shown that after a varying delay (usually, 30-60 minutes), brief laser stimuli above the threshold of both Aδ and C nociceptors applied to the skin innervated by the nerve no longer elicit a sensation of first pain, but still elicit a sensation of second pain. Furthermore, the Aδ fiber-related LEPs disappear and, most interestingly, ultra-late LEPs appear within a latency range compatible with the conduction of C fibers, i.e. approximately one second after stimulus onset [9,69].
A second method takes advantage of the difference in thermal activation threshold between heat-sensitive Aδ and C fiber receptors [32,59]. The thermal activation threshold of type II Aδ-fiber mechano-heat nociceptors (AMH Type II), which are thought to underlie LEPs related to the activation of Aδ nociceptors, lies in the range of 46°C. In comparison, heat-sensitive C fiber mechano-heat nociceptors are thought to have a significantly lower thermal activation threshold, lying in the range of 41°C [101]. Furthermore, C warm receptors can respond to increases in skin temperatures of less than 1°C above baseline skin temperature [50]. Exploiting these differences, Magerl et al. [59] devised an ingenious protocol based on a feedback controlled CO2 laser stimulator. At a base temperature of about 33°C, the skin was exposed to two successive heat ramps at 5-s interval. A first heat ramp of 50°C/s lasting 150 ms brought the skin temperature to 40°C, allowing the activation of heat-sensitive C nociceptors. Skin temperature was kept constant at 40°C for an additional 5 seconds and, then, the skin was briefly exposed to a second heat ramp bringing skin temperature to 48°C, allowing this time the activation of Aδ nociceptors. The first heat ramp elicited an ultra-late laser-evoked potential whose latency was compatible with the conduction velocity of C fibers, while the second heat ramp elicited a response with a shorter latency, compatible with the conduction velocity of Aδ fibers. Using a similar approach, it was recently shown that the use of temperature-controlled CO2 laser stimuli can be used to activate C-fiber afferents in a selective fashion and, most importantly, can be used to obtain reliable C-fiber LEPs [45].
A third method takes advantage of the fact that specific pathologies can lead to a selective impairment of myelinated nerve fibers. For example, Lankers et al. [51] observed ultra-late laser-evoked brain potentials in a patient with hereditary motor and sensory neuropathy type I, indicating the preservation of unmyelinated C fiber function. Similar anecdotal observations have been made in peripheral neuropathies characterized by a selective loss of Aδ fiber function (e.g. [12,111]).
Finally, one can exploit the fact that, in the skin, the density of C fiber free nerve endings is greater than the density of Aδ fiber free nerve endings. Indeed, depending on the species and methods for quantification, the density distribution of C fiber free nerve endings is about 2-8 /mm2, while the density distribution of Aδ fiber free nerve endings is thought to be <1 /mm2 [71,92]. Therefore, if a laser stimulus is applied using a very small stimulus surface area (e.g. 0.15 mm2) [8], there is a high probability that the beam will heat C fiber free nerve endings without concomitantly heating Aδ fiber free nerve endings. As compared to other methods, the approach has the advantage of not being invasive, and not requiring to lower the energy density of the stimulus. Several studies [8,19,20,72–74,86,110] have successfully used this approach to record ultra-late laser evoked potentials. Very recently, it was suggested that, using laser stimuli of varying beam diameters, the technique could actually be used to assess intra-epidermal nerve density non-invasively [67].
Whatever the method used, all of these studies have confirmed that when the activation of Aδ fiber nociceptors is avoided, or when the peripheral Aδ fiber afferent volley is blocked at peripheral level, the selective activation of C fibers elicits an ultra-late negative positive complex whose latency (750-1150 ms after stimulation of the hand) is compatible with the conduction velocity of unmyelinated C fibers. The morphology and scalp topography of these ultra-late responses very much resembles the morphology and scalp topography of LEPs elicited by Aδ fibers. The most prominent component of the response also consists of a negative-positive complex, maximal at the vertex, and often referred to as ultra-late N2-P2 [80]. Valeriani et al. [105] reported that, in addition to eliciting ultra-late N2 and P2 components, the selective activation of C nociceptors could also elicit an earlier negative component, labeled ultra-late N1. This ultra-late N1 would be similar to the N1 component preceding the Aδ-fiber N2-P2 complex. Indeed, such as the Aδ-related N1, the C-fiber related N1 was described as a peak displaying a lateralized scalp topography, maximal over the temporal region contralateral to the stimulated side.
A small number of studies [18,41,75] have applied source analysis methods to C-fiber LEPs. Such as Aδ-fiber LEPs, C-fiber LEPs are best explained by sources originating from bilateral opercular structures, as well as the anterior cingulate cortex [29]. Hence, it would appear that Aδ and C fiber afferent volleys elicit brain responses within the same structures.
LEPS MAGNETIC FIELDS
The brain responses elicited by thermal stimulation of the skin have also been studied using MEG [109]. Comparing laser-evoked brain responses recorded using EEG to those recorded using MEG could be interesting as the information recorded by both techniques may be complementary [33]. Indeed, deep sources of electro-cortical activity are thought to contribute very little to MEG signals, while superficial sources could be more accurately and more reliably captured using MEG. In addition, it is often stated that EEG signals mainly reflects sources of electro-cortical activity that are radial relative to the scalp surface, whereas MEG signals mainly reflects sources of electro-cortical activity that are tangential relative to the scalp surface. Finally, it has also been suggested that MEG recordings are more spatially accurate than EEG recordings because EEG recordings are blurred and distorted by the interposed layers [33]. However, it is important to highlight that these assertions have been questioned by several researchers [2,60,61].
Laser-Evoked Magnetic Fields Elicited by the Co-Activation of Aδ and C Nociceptors
Supporting the view that EEG and MEG provide information that is not redundant is the fact that the scalp topography and time course of laser-evoked magnetic fields is not identical to the scalp topography and time course of LEPs. Indeed, laser-evoked magnetic fields mainly consist of an early response peaking at 150 ms when stimulating the hand dorsum and 200 ms when stimulating the foot dorsum [46], whose latency is similar to the laser-evoked N1 wave identified using EEG. In other words, it seems that MEG is very sensitive to the electro-cortical activity reflected in the N1 wave of LEPs (and possibly other sources contributing at that latency), but is not very sensitive to the electro-cortical activity reflected in the later N2 and P2 waves of LEPs.
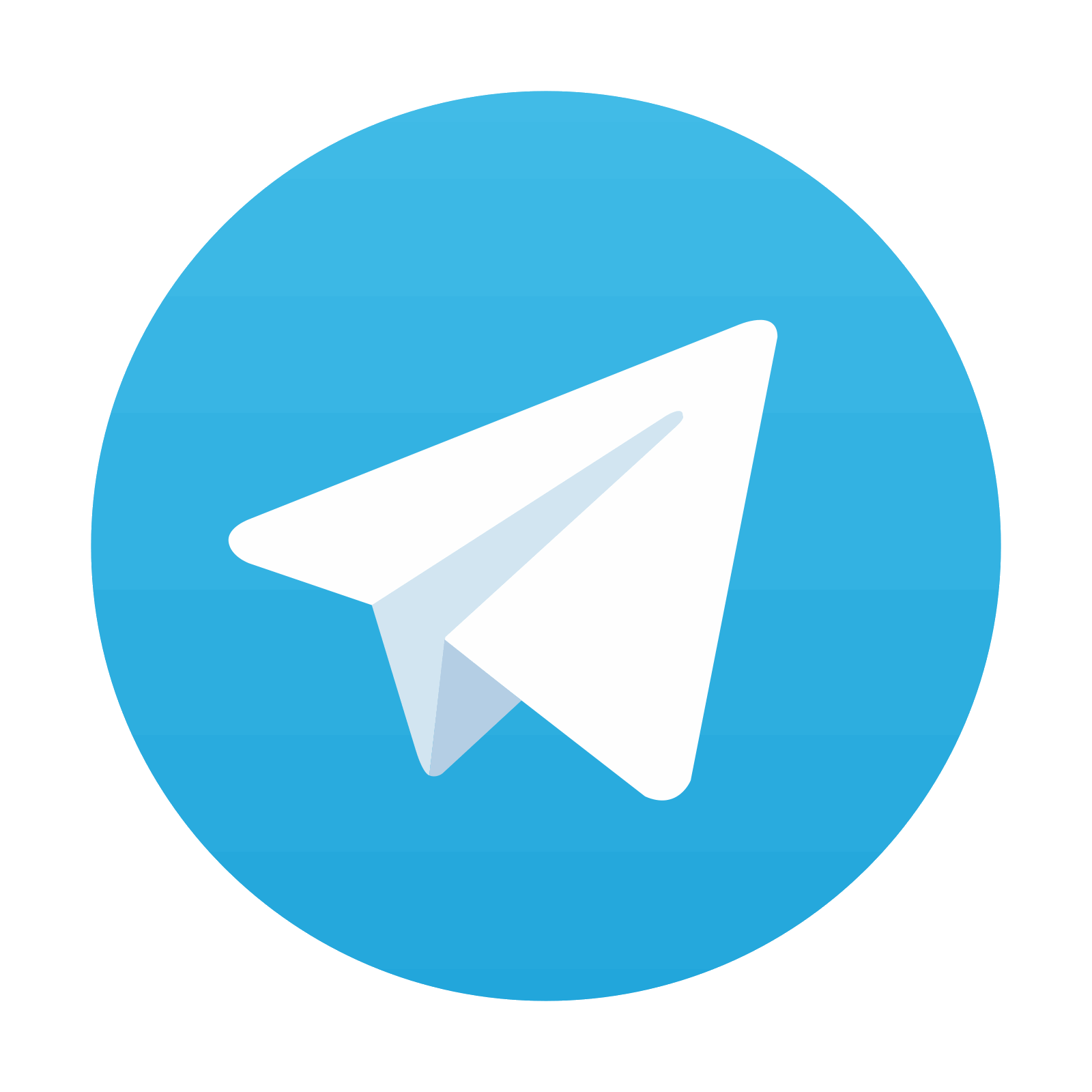
Stay updated, free articles. Join our Telegram channel
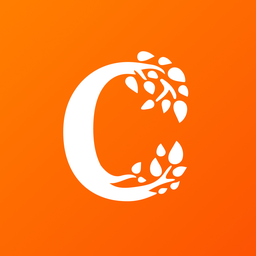
Full access? Get Clinical Tree
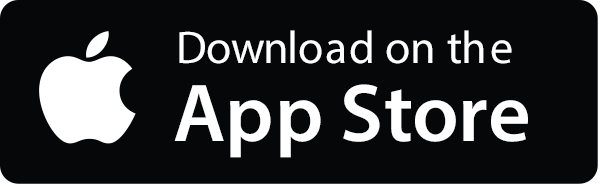
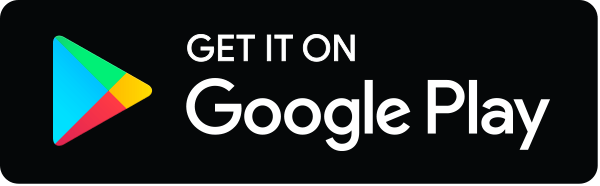