The HPA axis is one of the major organ systems regulating sodium balance and serum osmolality. Serum osmolality is sensed by osmoreceptors in the organum vasculosum of the lamina terminalis (OVLT) , located in the portion of the hypothalamus outside of the blood-brain barrier. Changes in osmolality of as little as 1 % are sensed by the osmoreceptors, which send projections to the supra-optic (SON) and para-ventricular (PVN) hypothalamic nuclei. The SON and PVN synthesize anti-diuretic hormone (ADH) and send projections to the posterior pituitary. These projections in the posterior pituitary release ADH into systemic circulation. ADH acts on the medullary collecting duct of the nephron, and causes increased water absorption. This increase in water absorption leads to a subsequent decrease in serum sodium concentration, thus driving down serum osmolality.
The other major system regulating sodium balance is the renin-angiotensin system (RAS) . Decreased sodium delivery to the macula densa triggers the synthesis and release of renin from the juxtaglomerular apparatus. Renin converts angiotensinogen to angiotensin I, which is then rapidly converted into angiotensin II by angiotensin converting enzyme (ACE) . Angiotensin II acts directly on the vasculature to increase systemic vascular resistance (SVR), increase ADH release, and stimulate the release of aldosterone from the zona golmerulosa of the adrenal cortex. Aldosterone acts on the distal tubules and collecting ducts of the nephron to increase the reabsorption of water and sodium, and increase the secretion of potassium. Abnormalities in either of these systems – HPA or RAS – can lead to disorders of sodium homeostasis.
Hyponatremia
Given that sodium and serum osmolality are tightly linked, and that osmolality is influenced by relative volume status, disorders of sodium homeostasis are categorized by intravascular volume status. Hyponatremia can occur in the context of intravascular hyper-, hypo-, or euvolemia, and patients’ intravascular volume status must be evaluated in order to diagnose the underlying cause of the hyponatremia.
Hypovolemic causes are due to the loss of both sodium and total body water as occurs with profuse sweating, prolonged vomiting or diuretic use. A rare cause of hypovolemic hyponatremia is cerebral salt wasting syndrome (CSW) . CSW is typically seen in the context of head trauma, or intracranial lesion, and is characterized by decreased renal sodium retention, polyuria, and dysautonomia. Treatment of hypovolemic hyponatremia is mainly supportive and aimed at fluid and electrolyte replacement. Fludrocortisone can be used to increase renal sodium reabsorption. Most cases typically resolve spontaneously within 2–4 weeks, although a few persist longer.
Hypervolemic hyponatremia is due to increased total body water, and typically occurs in the context of decreased effective serum osmolality, and is often associated with peripheral edema. Common etiologies include heart failure, nephrotic syndrome, and liver disease with associated ascites. In these states, plasma osmolality is decreased and oncotic pressure is unable to retain volume in the intravascular space. This leads to decreased effective circulating volume and the body responds by increasing water reabsorption, further decreasing serum osmolality. Treatment for these causes is aimed at the underlying etiology, and usually includes fluid restriction. A new class of medication, the vaptans, act as vasopressin antagonists and may find clinical use in some of these disease states.
Euvolemic hyponatremia is typically caused by either presence of osmotically active molecules (pseduohyponatremia) or excess secretion of ADH. Pseudohyponatremia occurs when hyperosmolarity from non-sodium osmotically active agents (glucose, triglycerides, immunoglobulins) draws water from the intracellular compartment into the intravascular space with subsequent dilution of sodium content. Syndrome of Inappropriate Anti-Diurectic Hormone (SIADH) is the paradigm for euvolemic hyponatremia, and is seen in a wide array of clinical conditions ranging from intracranial abnormalities to various tumors. SIADH is characterized by inappropriately elevated levels of ADH in the absence of a physiologically appropriate stimulus (i.e. hypotension or hypotonicity). The hallmark of SIADH is inappropriately concentrated urine relative to plasma osmolality. As discussed, one of the physiologic effects of ADH is water retention and these patients develop a pure water excess. Water is not confined to one fluid compartment, it distributes equally throughout all of them, thus these patients appear clinically euvolemic. Classically, water restriction has been the mainstay of SIADH treatment. In cases where water restriction is poorly tolerated, demeclocycline has been used. Demeclocycline is a tetracycline analogue that inhibits vasopressin mediated water reabsorption in the collecting duct. The new class of vasopressin inhibitors, the Vaptans, promises to radically alter the treatment of SIADH.
The deleterious physiologic effects of hyponatremia develop when sodium levels drop enough to significantly lower plasma tonicity, leading to a shift of water into the intracellular space. This leads to cellular dysfunction, particularly in the CNS and accounts for the clinical manifestations of confusion, coma and seizures. In cases of symptomatic hyponatremia, sodium repletion maybe given either orally, or in the form of concentrated 3 % saline. Correction must be gradual, so as to avoid neurologic devastation through central pontine myelinosis, which results from the rapid shift of water out of the intracellular space in the CNS. Plasma sodium concentration should not be raised by more than 0.5 mmol/L/h, and no more than 10 mmol/L in 24 h.
Hypernatremia
Hypernatremia leads to an increase in serum osmolality, with a subsequent shift of water out of the intracellular compartment and cellular dysfunction. As with hyponatremia, the CNS is particularly sensitive to these shifts and patients with symptomatic hypernatremia typically present with weakness, confusion and lethargy. In contrast to hyponatremia, hypernatremia is almost always a result of water loss in excess of sodium loss rather than excess sodium intake relative to water intake. As such, these patients are typically hypovolemic.
Most commonly, hypernatremia occurs via either evaporative losses or by excretion of large volumes of very dilute urine. Evaporative, or insensible losses result from evaporation of pure water from the respiratory tract or other exposed mucosal surfaces. These losses are highly variable and are increased by patients who are febrile, tachypnic, or in warm environments. Surgical procedures that expose large body cavities to room air greatly increase the surface area for evaporative loss, and can cause losses triple to those under normal environmental conditions. The loss of large volumes of dilute urine is characteristic of diabetes insipidus (DI) . DI results from either a lack of vasopressin release from the posterior pituitary, or lack of renal response to circulating vasopressin. Patients with DI who are able to regulate their water intake typically do not develop hypernatremia, as their input matches their output. However, patients who are unable to do so can rapidly develop hypernatremia and dehydration.
Treatment of hypernatremia depends on the underlying cause. In patients with central DI, a synthetic vasopressin analogue, desmopressin or DDAVP, is the treatment of choice. DDAVP lacks the hypertensive effects of vasopressin and can be given nasally, intravenously, or orally. In patients with nephrogenic DI, thiazide diuretics have a paradoxic anti-diuretic effect and are the treatment of choice if the underlying cause cannot be reversed. In patients who are hypernatremic secondary to free water loss, the correction of the free water deficit results in correction of the hypernatremia. Free water deficit can be calculated from the following equation:
![$$ \begin{array}{l}\begin{array}{l}\mathrm{Deficit} = \mathrm{T}\mathrm{B}\mathrm{W}\ \left(1-\left[140/\mathrm{Plasma}\ \mathrm{N}\mathrm{a}+\right]\right)\mathrm{where}\ \mathrm{T}\mathrm{B}\mathrm{W}\ \\ {}\mathrm{is}\ \mathrm{t}\mathrm{o}\mathrm{t}\mathrm{a}\mathrm{l}\ \mathrm{body}\ \mathrm{water}\ \left(\mathrm{L}\right)\ \mathrm{estimated}\ \mathrm{a}\mathrm{t}0.5 \times \end{array}\hfill \\ {}\begin{array}{l}\kern4em \mathrm{l}\mathrm{ean}\ \mathrm{body}\ \mathrm{weight}\ \mathrm{f}\mathrm{o}\mathrm{r}\ \mathrm{women}\kern0.5em \mathrm{a}\mathrm{nd}\\ {}0.6 \times \mathrm{l}\mathrm{ean}\ \mathrm{body}\ \mathrm{weight}\ \mathrm{f}\mathrm{o}\mathrm{r}\ \mathrm{men}.\end{array}\hfill \end{array} $$](/wp-content/uploads/2016/09/A161280_2_En_14_Chapter_Equb.gif)
![$$ \begin{array}{l}\begin{array}{l}\mathrm{Deficit} = \mathrm{T}\mathrm{B}\mathrm{W}\ \left(1-\left[140/\mathrm{Plasma}\ \mathrm{N}\mathrm{a}+\right]\right)\mathrm{where}\ \mathrm{T}\mathrm{B}\mathrm{W}\ \\ {}\mathrm{is}\ \mathrm{t}\mathrm{o}\mathrm{t}\mathrm{a}\mathrm{l}\ \mathrm{body}\ \mathrm{water}\ \left(\mathrm{L}\right)\ \mathrm{estimated}\ \mathrm{a}\mathrm{t}0.5 \times \end{array}\hfill \\ {}\begin{array}{l}\kern4em \mathrm{l}\mathrm{ean}\ \mathrm{body}\ \mathrm{weight}\ \mathrm{f}\mathrm{o}\mathrm{r}\ \mathrm{women}\kern0.5em \mathrm{a}\mathrm{nd}\\ {}0.6 \times \mathrm{l}\mathrm{ean}\ \mathrm{body}\ \mathrm{weight}\ \mathrm{f}\mathrm{o}\mathrm{r}\ \mathrm{men}.\end{array}\hfill \end{array} $$](/wp-content/uploads/2016/09/A161280_2_En_14_Chapter_Equb.gif)
Rapid correction of hypernatremia can lead to cerebral edema, therefore no more than half of the deficit should be replaced in the first 24 h of treatment. The remainder can be corrected in the following 24–48 h. Chronic hypernatremia is remarkably well tolerated secondary to compensatory changes which occur at the cellular level. These changes involve intracellular production of osmotically active substances to offset the extracellular hypertonicity.
Potassium
Potassium is the second most common cation in the body following sodium. Ninety-eight percent of the total body potassium content is contained intracellularly. The significant gradient in potassium concentration across the cell membrane leads to a negative electric charge in the intracellular space. This charge, known as a resting membrane potential , is necessary for the proper functioning of excitable tissues such as muscle and nerve. This gradient is maintained by active ion transport pumps located in the cellular membrane, which can account for up to 2/3 of a cell’s total energy expenditure. Small changes in extracellular potassium concentration can lead to dysfunction of excitable tissue with potentially catastrophic consequences. Maintenance of potassium homeostasis is accomplished at both the cellular and organ system level; shifts of potassium into and out of cells buffers against acute changes in extracellular potassium, while the renal system maintains a balance between potassium intake and excretion. Multiple disease states affect these systems and can lead to disorders of potassium regulation.
Hyperkalemia
Hyperkalemia maybe classified by duration of the insult as either acute (<48 h) or chronic. Acute hyperkalemia is almost always caused by a shift of potassium out of cells, but rarely can be caused by an excessive intake of potassium. A transcellular shift of only 2 % of intracellular K+ would cause serum K+ levels to double. Dramatic transcellular shifts of potassium are often associated with cell death, such as tumor lysis syndrome or rhabdomyolysis. A metabolic acidosis also results in a transcellular shift of potassium, as H+ displaces K+ from the intracellular compartment. Certain medications are also associated with transcellular shifts of potassium, notably digitalis and succinylcholine. Under normal circumstances, succhinycholine induced fasculations cause a small amount of K+ to leak from muscle. This small leak causes an increase in serum K+ by about 0.5 mmol/L. However, in patients with prolonged immobilization or paralysis, severe burns, or muscle inflammation this K+ leak can be significantly larger, with potentially fatal consequences. These diseases are associated with proliferation of immature forms of the AchR outside of the motor end plate. These extra receptors are activated by succinylcholine, resulting in more potassium being release from the cells than under normal circumstances. Excessive potassium intake is almost always iatrogenic, as can occur with overly aggressive oral or IV potassium replacement therapy. Banked blood contains a small amount of potassium, however massive transfusions can lead to accumulation of large amounts of potassium and cause symptomatic hyperkalemia. Causes of chronic hyperkalemia include renal failure, Addison’s disease, and both aldosterone deficiency and tubular unresponsiveness to aldosterone. Hyperkalemia causes depolarization of cardiac myocytes resulting in dysrhythmias and impaired conduction disorders. Classic EKG changes associated with progressive hyperkalemia include (in order of their appearance): peaked T waves, prolongation of the PR interval, widening of the QRS complex, loss of P wave, “sine wave” appearing ventricular fibrillation, and finally asystole. Hyperkalemia is also associated with paresthesias and skeletal muscle weakness progressing to a flaccid paralysis. Therapy for hyperkalemia is aimed primarily at stabilizing the cell membranes of excitable tissues. Once this has been achieved, therapy is guided towards redistribution of K+ into cells and enhanced elimination of K+ from the body. Calcium directly antagonizes the myocardial effects of hyperkalemia and is the treatment of choice for membrane stabilization. Redistribution of potassium into cells can be accomplished by both insulin and albuterol. A dextrose infusion should be started to counteract the hypoglycemic effects of insulin therapy in these patients. Increased elimination of potassium from the body can occur via either the renal or GI route. Renal elimination of potassium is increased by increased flow through the distal nephron, typically accomplished by administration of saline, and is enhanced by loop diuretics. GI losses of potassium are increased by the administration of sodium polystyrene sulfonate (SPS). SPS is a cation exchange resin that exchanges sodium for secreted potassium in the colon. SPS causes constipation and should be given with a cathartic. Cases of colonic necrosis have been reported following administration of SPS, with an estimated incidence of 1.8 % in post-operative patients according to a retrospective analysis. Hemodialysis can also be used to enhance potassium elimination.
Hypokalemia
As with hyperkalemia, hypokalemia may be classified according to duration as either acute (<48 h) or chronic. Acute changes are almost always caused by transcellular shifts of potassium into cells. This inward shift is often seen with treatment of DKA secondary to insulin therapy, and can also be seen in re-feeding syndrome due to increased endogenous insulin production. Other medications, such as β2-agonists, also cause a shift of potassium into cells and can cause acute hypokalemia. Chronic hypokalemia is usually the result of either decreased intake or increased elimination. Elimination can occur from either the GI or renal route. Renal losses of potassium are increased by diuretics, various antibiotics, and mineralcorticoids. Hypomagnesemia is also associated with renal K+ wasting, and often must be repleted along with potassium. Various inborn tubular transport abnormalities, such as Barter, and Gitelman’s syndromes are also associated with increased renal losses of K+ and hypokalemia. Hypokalemia leads to cell membrane hyperpolarization, and can cause cardiac arrhythmias and conduction defects. Non specific EKG changes include ST segment depression, T wave flattening and prominent U waves. Neuromuscular signs include weakness, muscle fatigue and myalgias. Treatment of hypokalemia involves replacement of the body deficit and correction of underlying cause when able. Potassium may be given either orally or IV. If given IV, potassium should be administered over 1–2 h to avoid causing a hyperkalemia. Serum potassium levels peak immediately following an infusion, and over the next 2–3 h decrease to the new steady state. Repeat measurements of serum potassium should be taken after the new steady state has been achieved to further guide therapy.
Chloride
Chloride is the predominant anion in the extracellular fluid. As mentioned above, electroneutrality is maintained at all times; that is, the concentration of cations and anions is equal and charges offset. As such, changes in chloride concentration significantly effect the concentration of other anions such as bicarbonate, lactate and other organic acids. Many of these other anions take part in the buffering of serum H+ concentration and help determine plasma pH. Due to the interplay of chloride and other serum anions, chloride physiology and acid base balance are closely related and interdependent.
An example of this sort of interdependence can be seen in patients who have been resuscitated with large volumes of normal saline. 0.9 % normal saline contains 154 meq of sodium and 154 meq of chloride compared to a normal serum chloride concentration of approximately 100 meq. This increase in serum chloride causes a reciprocal decrease in other serum anions, notably bicarbonate. As serum bicarbonate levels decrease, there is a concomitant increase in unbuffered serum H+ concentration and serum pH decreases resulting in an acidosis. The role of chloride physiology and acid-base balance will be further discussed in the next section.
Introduction to Acid Base Physiology
The tight control of extracellular hydrogen ion concentration is of paramount importance to the function of trans-membrane ion transport pumps and intracellular biochemical reactions. As such the body has developed numerous weak acid buffer systems to maintain a homeostatic pH between 7.35 and 7.45. Deviations in pH beyond this zone are termed acidemia (pH <7.35) and alkalemia (pH >7.45). The presence of acidemia or alkalemia indicates gross metabolic or respiratory abnormalities, which if uncorrected, may lead to end organ dysfunction and death.
Acid-Base balance is a complex physiochemical process. Two different approaches can be used to explain acid-base interaction: anion gap/base excess and strong ion difference (Stewart approach). The Stewart approach introduced by Peter Stewart in 1981 emphasizes two important elements of physical chemistry as the driving forces for acid base balance: electroneutrality and conservation of mass. The primary tenant of Stewart’s approach is that serum bicarbonate does not alter blood pH. According to Stewart’s theory, pH is the result of the interplay of three variables: Strong Ion Difference (SID) , PaCO2, and plasma concentration of weak non-volatile acids such as albumin and phosphate (Atot). The SID equals the difference between completely dissociated cations and anions in plasma. The equation for SID consists of the most abundant ions in plasma and is calculated as such:
Processes that increase the SID cause an alkalemia, whereas processes that reduce it cause acidemia. For example, the loss of gastric fluid, which has a high concentration of Cl−, increases the SID and thus leads to alkalemia; by comparison, infusion of sodium chloride, a solution with equal parts Na+ and Cl− thus an SID of zero, reduces the SID and causes an acidemia.
![$$ \begin{array}{l}\mathrm{S}\mathrm{I}\mathrm{D} = \left(\left[{\mathrm{Na}}^{+}\right] + \left[{\mathrm{K}}^{+}\right] + \left[{\mathrm{Ca}}^{2+}\right] + \left[{\mathrm{Mg}}^{2+}\right]\right)\\ {}-\left(\left[{\mathrm{Cl}}^{-}\right] + \left[{\mathrm{Lactate}}^{-}\right]\right) = 40-44\ \mathrm{mEq}\end{array} $$](/wp-content/uploads/2016/09/A161280_2_En_14_Chapter_Equc.gif)
The anion gap approach is based on the Bronsted–Lowry definition of an acid in which the primary extracellular buffer system is the equilibrium of carbonic acid and bicarbonate represented by the equation below.
In this equation αCO2 represents the solubility coefficient of CO2 (0.03) and pK represents the equilibrium constant (6.1). A derivative of this equation, known as the Henderson equation , simplifies matters as such:



From this equation, it is evident that changes in pH may be either the result of a change in pCO2 (referred to as respiratory) or HCO3 − (referred to as metabolic). There is much emerging evidence of the likely clinical utility of the Stewart approach and while understanding both approaches is ultimately important, due to its simplicity and widespread use, the anion gap approach will be discussed in this chapter.
Maintenance of homeostatic concentrations of CO2 and HCO3 −, including compensatory responses to insult, is a result of the interplay of the pulmonary and renal systems. Consequently, metabolic disturbances are accompanied by a respiratory compensation and visa versa. Compensation results in normalization of pH and can give some indication as to the duration of the insult, with chronic processes being better compensated. Attention must be paid to compensation, as multiple acid-base abnormalities can co-exist in the same patient, which are diagnosed by comparison of anticipated and actual compensatory changes. More than one metabolic abnormality may be present in a patient at one time, however, only one respiratory disturbance is possible at any given moment.
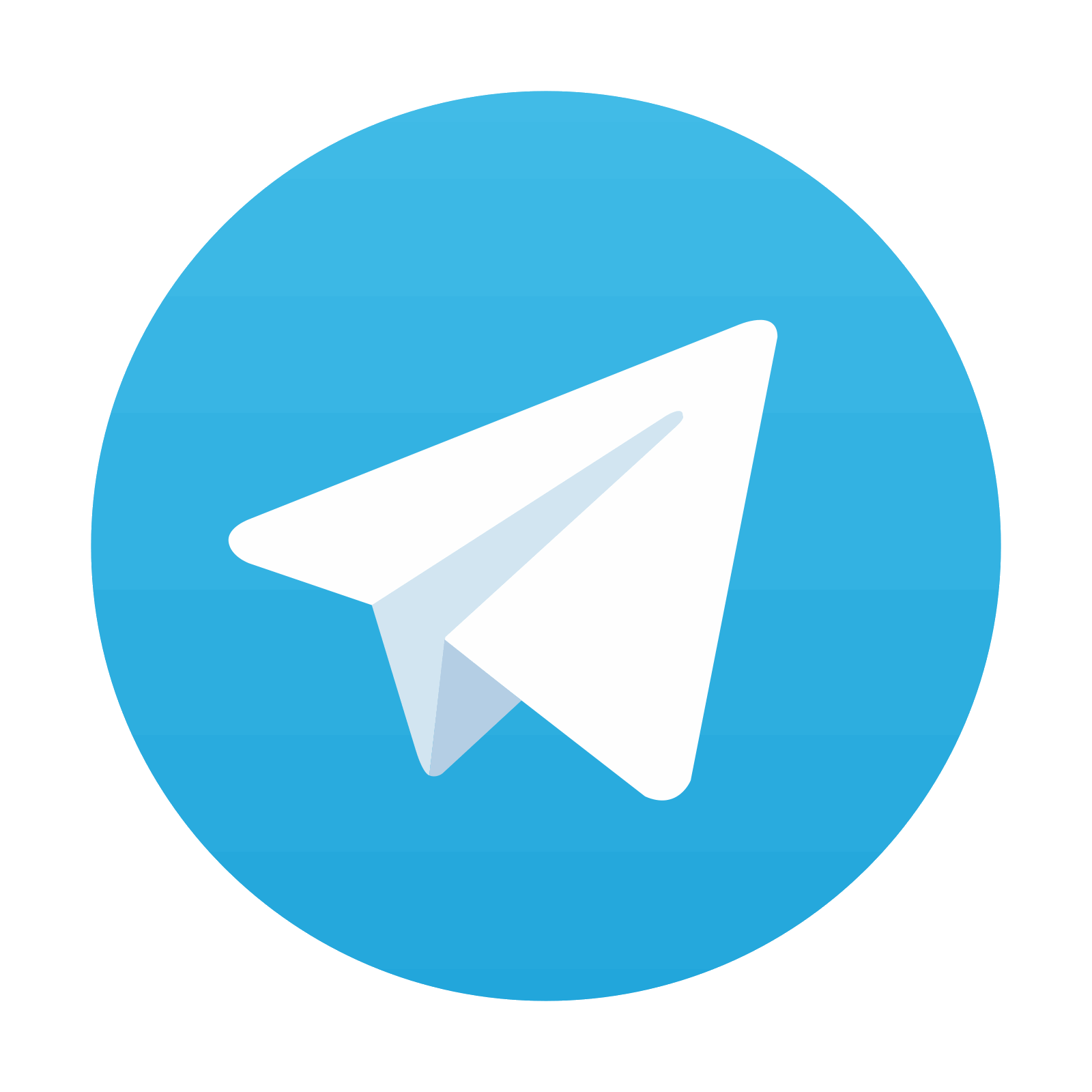
Stay updated, free articles. Join our Telegram channel
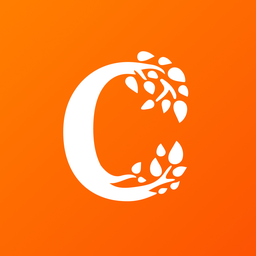
Full access? Get Clinical Tree
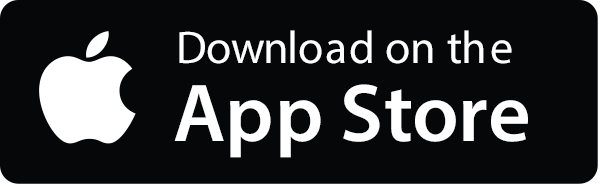
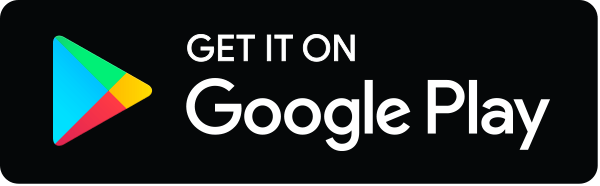