Diagnostic and Prognostic Neural Blockade
Quinn H. Hogan
Stephen E. Abram
The use of neural blockade to establish the diagnosis or prognosis of painful conditions is motivated by several compelling characteristics of clinical pain treatment: Pain is an entirely subjective phenomenon with limited means of measurement; painful conditions are inexactly delineated, so that a single descriptive label may be applied to a heterogenous group of maladies, and our understanding of the pathophysiology of pain is poorly developed; and, finally, chronic pain is the result of nociception (the stimulation of pain-sensing pathways), sensory modulation (segmental and descending inhibition, descending facilitation, glial and neuronal sensitization), and neuropathy (aberrant neural function), as well as social, emotional, behavioral, financial, and legal influences. The ambiguity caused by these factors argues strongly for the use of neural blockade to gain clear knowledge of the pathophysiology of the pain, specifically the contribution of sympathetic activity, the site of nociception (visceral versus somatic), or the pathway of afferent neural signals (in an extensively injured limb for example). Information gained from neural blockade can be a useful guide toward choice of medicines, therapeutic blocks, or surgical therapy. Also, neural blockade may be used to anticipate response to surgical ablation, which rarely produces a more satisfactory response than does the prognostic block. By means of suitable neural blockade, the patient may experience motor and sensory changes on a temporary basis before committing to a long-term or permanent lesion.
However, the same uncertainties surrounding pain treatment that lead clinicians to seek hard data from neural blockade techniques also cloud the information gained from them. It is rarely simple to interpret even the most meticulously performed procedure. We may unwittingly confirm preconceived expectations or, at worst, respond to the disproportionate remuneration associated with performing procedures. Few blinded and controlled studies exist that test the utility of these alluring methods.
The premise of this chapter is that neural blockade techniques are informative only in proportion to the care with which they are performed and the thoroughness with which the response is evaluated, and that the findings should be interpreted cautiously. Therefore, we emphasize limitations, so that the reader may avoid the pitfalls. Physiologic, anatomic, and psychosocial issues that influence the quality of information from diagnostic blocks are reviewed first, after which the various procedures are discussed.
Pain Physiology
The rationale for performing diagnostic neural blockade often is based upon the same misconception that prompts surgeons to interrupt neural pathways for permanent pain relief, namely the belief that pain is transmitted by a simple and direct “hard-wired” system. This theory, based on the Cartesian model of pain perception conceived in the 17th century, dominated thinking about pain sensation through the mid 20th century and continues to drive much present-day treatment. Use of a nerve block to identify a nerve pathway that is the source of an individual’s ongoing pain assumes three potentially false premises: (a) pathology causing pain is located in an exact peripheral location, and impulses from this site travel via a unique and consistent neural route; (b) injection of local anesthetic totally and selectively abolishes sensory function of intended nerves; and (c) relief of pain following local anesthetic block is due solely to blockade of the targeted neural pathway. These assumptions are limited by certain complexities of the anatomy, physiology, and psychology of pain perception, and the effect of local anesthetics on impulse conduction (see Chapters 2 and 31,32,33,34,35,36).
Nociceptor Activity
Pain that originates in somatic structures is generally associated with activation of nociceptors, sensory receptors that respond to intense, potentially tissue-injuring, stimulation (Table 38-1). There is considerable controversy about the existence of visceral receptors that respond solely to pain, and many researchers argue that visceral pain results from high-frequency discharge of visceral afferents that ordinarily subserve other functions (1,2). In somatic structures, frequency of discharge of nociceptors correlates directly with the intensity of the stimulus. Thermal nociceptors, for instance, increase their discharge frequency in a linear fashion as temperature rises above 43°C (3). This schema is compatible with the Cartesian “hard-wired” model.
However, receptor performance is not a fixed function of stimulation, but is also sensitive to tissue factors (see Chapter 31, Figs. 31-2,31-3,31-4). Bradykinin, histamine, and 5-hydroxytryptamine (5HT; serotonin) are capable of lowering response thresholds of nociceptors (4). Several eicosanoids are important in the sensitization of nociceptors, and at least two of the prostaglandins (PGE2 and PGF2α) increase nociceptor sensitivity, whereas prostacyclin potentiates edema induced by bradykinin and histamine.
The transient receptor potential channel, vanilloid subfamily member 1 (TRPV1) has been identified as an important skin nociceptor, and is well characterized in the basic science literature (5). This receptor is activated extracellularly by heat, acids, and chemicals with a negative electrical charge, and
intracellularly by arachidonic acid products of the lipoxygenase pathway such as anandamide and N-arachadonoyl-dopamine (NADA). Tissue inflammation markedly lowers the TRPV1 threshold to activation by heat (6). Other TRP channels appear to be candidates for cold pain transduction (TRPA1), and TRP channels as well as acid-sensing ion channels (ASICs) may be involved in the initiation of mechanical nociception (6) (see Chapter 32).
intracellularly by arachidonic acid products of the lipoxygenase pathway such as anandamide and N-arachadonoyl-dopamine (NADA). Tissue inflammation markedly lowers the TRPV1 threshold to activation by heat (6). Other TRP channels appear to be candidates for cold pain transduction (TRPA1), and TRP channels as well as acid-sensing ion channels (ASICs) may be involved in the initiation of mechanical nociception (6) (see Chapter 32).
Table 38-1 Diagnostic blocks—limitations from Variable 1ry afferent nerve activity | ||
---|---|---|
|
Peripheral nerve activity associated with pain perception may arise from injured nerves independent of natural nociceptor activation. It is now well accepted that spontaneous impulses can be initiated from a neuroma or from an injured nerve segment (7,8), and there is evidence that dorsal root ganglia (DRG) proximal to injured or transected nerves participate in abnormal impulse generation (7). Blockade of a nerve proximal to the injured segment may not provide relief of pain if spontaneous activity continues more proximally at the level of the DRG. This may lead to the false assumption that the injured nerve has not initiated the patient’s pain (Table 38-1) (see Chapters 31 and 32).
Nerve blocks usually are interpreted in terms of their effect on afferent neural activity, but important efferent traffic must be considered. Impulse generation arising from an injured nerve fiber is likely to be propagated both orthodromically toward the spinal cord and antidromically toward the innervated tissues. For instance, bursts of sural nerve activity are recorded during straight leg-raising in a patient with S1 radiculopathy (9). Antidromic activity from injured sensory nerves (probably only C-fibers) is thought to cause release of substance P and perhaps other substances, such as bradykinin, histamine, 5HT, and prostaglandins, which may produce changes in nociceptor thresholds by direct and indirect means (10). Therefore, nerve block distal to the primary site of nerve pathology may alter pain perception by interrupting antidromic impulses, contrary to the common assumption that axonal function must be interrupted proximal to the area of injury to provide relief. Peripheral blockade of the sciatic nerve has been shown to provide profound relief of pain for patients with documented lumbosacral radiculopathy (11,12), perhaps by blocking those antidromic impulses that arise from the nerve root or DRG and are propagated to the periphery, thus producing changes in nociceptor sensitivity (13) (Table 38-1).
Sympathetic Contributions
Another important type of efferent traffic affected by neural blockade is sympathetic motor activity, which can alter responses in sensory fibers. Receptors at the terminus of C-fibers from an injured nerve become excited during sympathetic stimulation or norepinephrine application and show enhanced responsiveness to irritating stimuli (14). At the site of the nerve injury, sympathetic efferent impulses may depolarize nociceptive afferent fibers, potentially producing both orthodromic and antidromic activity. Increased sympathetic activity or high levels of norepinephrine have been shown to increase discharge rates of spontaneous impulses arising from neuromas (15,16), and the injection of epinephrine in the vicinity of neuromas in pain patients is associated with aggravation of pain (17) (Table 38-1).
In uninjured tissues, it is well accepted that sympathetic supply can modulate sensory responses (18), but the role of the mechanism in producing pain is less certain. Mechanoreceptor sensitivity is heightened by increases in sympathetic discharge rates. Since nociceptors are not excited by sympathetic stimulation, elevated sympathetic efferent activity alone is unlikely to cause pain. However, aberrant central processing of these signals by sensitized wide dynamic range (WDR) neurons in the dorsal horn (see later discussion) may result in the allodynia (18) present in certain cases of complex regional pain syndrome type 1 (CRPS-1) (Fig. 38-1). Following peripheral nerve injury, an increase occurs in the population of sympathetic nerve fibers in the adjacent DRG (19). Sprouting sympathetic fibers arise from the injured spinal nerve and spread into the DRG, creating basket-like structures surrounding cell somata. The functional changes associated with these plasticity changes have not been characterized, but it seems likely that alterations in the activity of these sympathetic efferents may alter the sensitivity of the neurons they contact (see Chapters 31 and 32).
Pain reduction following sympathetic blockade cannot clearly distinguish between several possible pain mechanisms, including irritation of peripheral nociceptor sensitized by sympathetic efferent activity, signal generation in a neuroma induced by normal sympathetic tone, primary pathologic increase in sympathetic activity resulting in abnormally sensitive receptors, and inflammatory or central effects. When local anesthetic blockade of the sympathetic chain produces temporary relief, surgical or neurolytic interruption of the sympathetic pathways can, nonetheless, fail to provide sustained analgesia. Upregulation of adrenergic receptors in the affected limb may lead to such receptor hypersensitivity that circulating catecholamines may be sufficient to reproduce sympathetically maintained pain.
Spinal Processing
Whatever the contribution of receptor, neuropathic, or sympathetic mechanisms, activity in nociceptive afferent fibers is subject to further, variable processing in the spinal cord (Table 38-2). The balance between large- and small-fiber inputs is an important determinant of the response of dorsal horn neurons to noxious stimulation (20). Conceivably, loss of large-fiber activity following peripheral or neuraxial blockade could increase dorsal horn cell activity, particularly if C-fiber input is preserved (see Chapter 35, Fig. 35-1), thus producing a paradoxical increase in pain.
In addition to segmental influences on dorsal horn function, descending pathways modulate the response of spinal cord neurons to sensory stimuli (see Chapter 31, Figs. 31-18 and 31-19). Noradrenergic and serotoninergic fibers originate in the medulla and descend via the dorsolateral funiculus of the spinal cord to terminate in the dorsal horn, where they inhibit nociceptive traffic (21). Since these tracts lie superficially in the cord, they are susceptible to blockade by intrathecally administered local anesthetics, possibly leading to disinhibition of nociceptive transmission. The relative effect of the drug on
afferent pathways, versus descending inhibitory tracts, would then determine the analgesic effect of a subarachnoid block. Descending cerebral influences may obscure findings during a diagnostic test by producing analgesia in response to stress, independent of the specific nature of the block. Conversely, descending modulation may be stimulatory and produce pain that is independent of sensory input. Dubner and co-workers (22) have demonstrated in primates that nonpainful signals (flashing light) can be associated with nociceptive stimuli (laser heat) by conditioning with repetitive simultaneous presentation. Eventually, the light alone can result in firing of secondary nociceptive neurons and presumably in the sensory experience of pain. The importance of this model is that diagnostic blocks that produce no relief may suggest a diagnosis of malingering or psychiatric disease, when in fact descending influences are generating sensory activity.
afferent pathways, versus descending inhibitory tracts, would then determine the analgesic effect of a subarachnoid block. Descending cerebral influences may obscure findings during a diagnostic test by producing analgesia in response to stress, independent of the specific nature of the block. Conversely, descending modulation may be stimulatory and produce pain that is independent of sensory input. Dubner and co-workers (22) have demonstrated in primates that nonpainful signals (flashing light) can be associated with nociceptive stimuli (laser heat) by conditioning with repetitive simultaneous presentation. Eventually, the light alone can result in firing of secondary nociceptive neurons and presumably in the sensory experience of pain. The importance of this model is that diagnostic blocks that produce no relief may suggest a diagnosis of malingering or psychiatric disease, when in fact descending influences are generating sensory activity.
Table 38-2 Diagnostic blocks—limitations from changes in spinal processing | ||
---|---|---|
|
Convergence and Referred Pain
Another feature of pain perception that may confound the application of diagnostic blockade is the phenomenon of convergence (Table 38-2). Many second-order neurons in the spinal cord receive a variety of inputs from primary afferents with either visceral or somatic receptive fields (23) (see Chapter 31, Figs. 31-20,31-21,31-22). In other instances, convergence is the result of individual C-fiber neurons that have both visceral and cutaneous collaterals (24). When afferent input arises from both somatic and visceral structures, or from separate somatic foci, the perception of pain may be dependent on a summed level of neuronal activity from both components (Chapter 31, Fig. 31-20). Interruption of one limb of the convergent inputs may be enough to provide complete pain relief, leading to false assumptions about the source of the pain. For example, a patient with pain of pancreatic cancer may have inputs from splanchnic nerves, as well as from a focus of myofascial pain in the paravertebral muscles. Infiltration of a painful trigger point in the affected muscle may reduce the combined input to a level insufficient to exceed the pain threshold, and the interpretation would be that the pain is entirely somatic. As a further example, a patient may have combined gluteus medius myofascial pain and S1 radiculopathy, with pain perceived in the distribution of the S1 root. Infiltration of the gluteal trigger point provides blockade of input from muscle afferents to the S1 segment, and may provide sufficient decrease in convergent input to relieve the radicular pain completely on a temporary basis. This could then be interpreted as providing evidence that the patient’s pain is entirely myofascial in origin, and the radicular component may be ignored when therapy is proposed.
Plasticity
Sensory processing is not stable but depends on preceding events, a phenomenon called neuronal plasticity (Table 38-3). Small-fiber (nociceptive) activity initiates a series of events in the dorsal horn that lead to heightened responsiveness of neurons activated by noxious stimuli. A brief noxious stimulus produces activation of the α-amino-3-hydroxy-5-methyl-4-isoxazopropionic acid (AMPA) receptor, which in turn produces a brief postsynaptic potential. Repetitive or prolonged firing of small afferent fibers (conditioning stimuli) releases excitatory amino acids, which in turn activates the N-methyl-D-aspartate (NMDA) receptor, causing prolonged postsynaptic potentials. NMDA activation gives rise to a series of intracellular biochemical events (Fig. 38-2) that produce enhanced neurotransmitter release from primary afferents and heightened sensitivity of postsynaptic neurons lasting many minutes to hours (25). In certain instances, more prolonged changes in sensory processing, known as long-term potentiation (LTP), may occur (25,26) (see also Chapter 31, Figs. 31-10,31-11,31-12,31-13,31-14,31-15,31-16 and Chapter 32).
Table 38-3 Diagnostic blocks—limitations from plasticity | |
---|---|
|
In addition to these neuronally mediated mechanisms of central sensitization, activation of glial cells in the central nervous system (CNS), which occurs in response to triggers to neuroimmune activation, such as nerve injury, intense nociceptor activation, inflammation, or infection, can also lead to increased responsiveness of pain projection neurons. The mobilization of microglia, which function as macrophages in the CNS, leads to the increased production and release of proinflammatory cytokines, such as interleukins (IL-1, IL-6) and tumor necrosis factors (TNF-α), which in turn increase the sensitivity of surrounding neurons. Subsequent activation of astrocytes prolongs these responses. The administration of exogenous opioids enhances glial activation, and can in fact directly produce glial activation, cytokine production and hyperalgesia in the absence of tissue or nerve injury (27).
Sensitization in response to noxious stimulation, nerve injury, or chronic opioid administration can affect WDR neurons, which ordinarily respond at very low firing rates to non-noxious (mechanoreceptor, proprioceptor, thermoreceptor) inputs and at high firing rates to nociceptor activity. Following sensitization or LTP, these cells may respond to non-noxious stimuli at sufficiently high firing rates to cause pain perception (allodynia). It is impossible to predict responses to local anesthetic blockade of afferent impulses under conditions of dorsal horn sensitization. Afferent blockade of conditioning stimuli could lead to normalization of dorsal horn responsiveness and profound, prolonged relief. In other circumstances, however, spinal sensitization might persist independently of afferent activity, with little or no change in pain level.
Pain and abnormal sensory responses after injury often are found in a distribution inconsistent with any nerve or root, such as over an entire limb, or in a stocking or glove pattern. This may lead to the diagnosis of psychoneurosis rather than to a neurologic condition. However, injury to a single peripheral nerve may create allodynia in adjacent territories innervated by other nerves, due to altered central processing of afferent signals from the uninjured as well as injured nerve (28). Blockade of the uninjured nerve will relieve pain within the borders of its innervation. The likely but erroneous interpretation would be that the blocked nerve had been injured, which could lead to injection therapy or surgical neurolysis.
Local anesthetic blockade may outlast the duration of local anesthetic effect by hours or days (29), leading to speculation that pain is psychosomatic or factitious. However, it is possible that a period of interruption of nociceptor activity may lead to temporary reversal of the sensitization of spinal cord neurons. Once the peripheral generator recommences, hours or days may go by before sufficient dorsal horn sensitization occurs to cause perception of pain.
Conversely, decreases in afferent input can lead to functional changes in the dorsal horn. Following periods of deafferentation, cells that respond to noxious stimulation become hypersensitive to remaining afferent inputs and may develop responsiveness to stimulation of tissues that did not previously produce activation (receptive field expansion) (30). Denervation may also produce sufficient sensitization of WDR neurons so that non-noxious stimulation, including stimuli from outside the original receptive field, can produce pain (see Chapters 31 and 32). Blockade of such stimulation could cause false indication of the pathology site. Alternatively, blockade of an injured nerve may not provide relief of pain and allodynia if the receptive field of sensitized dorsal horn neurons has spread beyond the distribution of the injured nerve, again leading to the mistaken conclusion that the injured nerve is not involved. Denervation of peripheral afferent fibers has been shown to
cause dramatic functional changes in the responses of WDR neurons in the dorsal horn (31).
cause dramatic functional changes in the responses of WDR neurons in the dorsal horn (31).
Summary
These physiologic observations demonstrate that pain is not a process taking place at a single site, is not fixed over time, and is not solely dependent on afferent activity. In addition to the diagnostic implications noted earlier, short-acting local anesthetic blocks often fail to predict beneficial responses to neurodestructive procedures. Nerve regrowth may lead to pain recurrence, but several other factors also cause failure of peripheral neuroablation, despite profound relief from local anesthetic blocks. The nerve injury itself, whether caused by surgical transection, neurolytic block with phenol or alcohol, thermocoagulation, or cryotherapy, may induce spontaneous discharge or increased mechanosensitivity at the site of injury (7,8,15,32). Successful denervation, even in the absence of increased peripheral inputs, may lead to changes in dorsal horn cell function, with sensitization of WDR neurons and expansion of receptive fields.
Local Anesthetic Physiology
Intensity of Blockade
Diagnostic and prognostic blocks are accomplished by the action of local anesthetics (and occasionally by neuraxial opioids [33]) upon nerves. It has long been recognized that neural blockade is not an all-or-none response. For instance, analgesia usually is evident earlier and to a greater extent than loss of perception of mechanical stimuli after peripheral neural blockade. This should be recalled when diagnostic sympathetic blocks are performed. The lack of anesthesia to touch in the involved area does not assure that pain relief is accomplished by sympathetic interruption, because a subtle somatic block could produce analgesia without anesthesia, resulting in pain relief independent of a sympathetic mechanism (34). In the opposite sense, apparent intense blockade with complete insensitivity to touch and pain is nonetheless not a complete afferent blockade. Studies of different types of blocks with various agents uniformly demonstrate incomplete elimination of somatosensory potentials evoked by stimulation of the anesthetized region (35). This may be the mechanism behind tourniquet pain and the humoral response to upper abdominal regional anesthesia, both of which occur despite apparently adequate sensory blockade. If pain continues after a diagnostic block, one cannot be certain that the injected pathway is not involved, since neural blockade is rarely complete.
The variable and partial nature of local anesthetic effects is evident also in blockade of efferent sympathetic activity. Skin conduction responses, a manifestation of sympathetic action at sweat glands, often is present in areas of apparently complete somatic blockade (36), and skin cooling has been noted in the center of a truncal band of segmental epidural anesthesia (37). During total thoracolumbar epidural anesthesia, norepinephrine levels decrease by only about 60% (38) or not at all (39), indicating persistent sympathetic synaptic release. These considerations weaken the predictive value of sympathetic blocks, unless monitoring confirms the loss of sympathetic activity concurrent with the onset of relief.
Table 38-4 Diagnostic blocks—limitations from Complexity of local anesthetic effect | |
---|---|
|
Differential Block (Table 38-4)
The variable effects of local anesthetics upon fibers performing different functions is termed differential block (Table 38-4). Were it possible to predict and control the neural modalities that are blocked, diagnostic distinctions could be made by selectively interrupting sympathetic or somatic fibers. This goal has proved elusive. The physiologic mechanisms that result in differential effects of local anesthetics are complex and multiple (40) (see also Chapter 2). Most commonly cited is the importance of fiber size. Since fibers of different cross-sectional areas serve different functions (large Aα → motor, Aβ touch/proprioception; small Aδ → cold/hot/pain, B → preganglionic sympathetic, C → postganglionic sympathetic, pain, temperature), dependence of anesthetic action upon size would explain clinically observed graded blockade of sensory and motor functions by local anesthetics. Erlanger and Gasser formulated this concept in 1929, but despite the appealing simplicity of the model, it has not withstood the test of time. They studied the effects of cocaine upon amphibian nerves, but only examined large myelinated A-fibers at room temperature and not under equilibrium conditions. Further study (41,42) has shown that, contrary to the size principle, the concentrations (CM) necessary for blockade of A- and B-fibers are less than those for C-fibers. In general, the intrinsic sensitivity of nerve fibers to local anesthetics is probably A <B <C. Problematic for the use of local anesthetics in diagnosis, however, is the great variability of sizes within a fiber type, and the lack of correlation of size and CM within the group (41,43). The overlap of CM between different groups “appears to negate any possibility of obtaining steady state differential interruption” by local anesthetics (41).
The size principle fails to explain the differential effects clinically evident during non–steady state conditions, but different diffusion barriers of the various fiber types probably does. The lipid barrier of myelinated A-fibers is a greater impediment to ability of local anesthetics to reach the axonal membrane binding site than it is for C-fibers, which lack the insulating myelin (44). Despite the inherent greater resistance of C-fibers to blockade, they are exposed to higher local anesthetic concentration early in the onset of the block, because of more rapid diffusion, so that the sequence of blockade is usually B first (due to intrinsic sensitivity), then C and Aδ before Aα and Aβ. Using intraneural recording to study conduction in radial nerves of human subjects after injection of 0.25% lidocaine (45), preferential blockade of C-fibers is evident. Full differential block is not possible, however, since completely abolished C-fiber activity is accompanied by partial A-fiber block. Sensory loss progresses in the order of sensibility for warmth, dull ache, cold, prick, and finally touch (46). The combination of
high lipid solubility and low pKa (high nonionized fraction) for etidocaine accounts for its thorough penetration into well protected Aα (motor) fibers, and therefore minimal differential block, compared to bupivacaine, which has a weak motor block (see Chapter 2).
high lipid solubility and low pKa (high nonionized fraction) for etidocaine accounts for its thorough penetration into well protected Aα (motor) fibers, and therefore minimal differential block, compared to bupivacaine, which has a weak motor block (see Chapter 2).
Even with concentrations of local anesthetic high enough to eliminate sodium (Na+) conductance completely, an action potential can still “jump” two adjacent totally blocked nodes (about 4 mm for the largest fibers) and excite the nerve beyond the blocked segment. To prevent conduction, at least three nodes in succession must be blocked (47) (see Chapter 2). If local anesthetic is limited in longitudinal extent, large fibers with long internodal distances may lack exposure to three nodes, whereas smaller fibers have the necessary three nodes exposed and are blocked (Fig. 38-3). At concentrations that produce less intense Na+ channel blockade, the influence of exposure length extends even further, so that a concentration of local anesthetic that blocks conduction in 3 cm of exposed nerve may not block conduction when only 2 cm is exposed (48). With low concentrations of local anesthetic, not all Na+ channels are inactivated, so a diminished but present action potential results (i.e., the action potential is not “all-or-none”). Less current reaches the adjacent node, causing it to fire but with an even smaller action potential. During this so-called decremental conduction, the action potential may propagate for many nodes before it finally fails to depolarize the next node. The result is that (a) conduction may fail through a segment of exposed nerve even if none of the nodes have been made completely inexcitable (Fig. 38-4), and (b) CM is inversely related to exposed nerve length. These phenomena may explain differential blockade that develops with spinal and epidural anesthesia (49), but also dictate that anesthetic potency and the degree of differential effects varies with the length of nerve exposed, an added variable that is hard to control (see also Chapter 2).
Further subtle influences upon local anesthetic action may cloud the interpretation of diagnostic blocks. Sodium channel closure by local anesthetics depends on nerve use; tonic block in an infrequently firing axon is less intense than the phasic block that develops progressively with higher firing rates. Local anesthetic will affect those fibers more completely that are most active. The ongoing activity of B vasomotor fibers may contribute to their preferential blockade, especially since these fibers show the greatest degree of phasic block. Phasic amplification of blockade may play a minor role for C (pain) fibers because their firing rates are too low. The spectrum of anesthetic effects will depend, therefore, upon the pattern of activity of the subject’s various neuron types when the diagnostic block is undertaken, and also will depend upon the choice of anesthetic. Bupivacaine, the agent with the greatest degree of phasic block, also shows a high degree of differential blockade. Since the earliest perturbation of nerve function at very low anesthetic concentrations is the prolongation of the latent interval for refiring (43), information encoded with bursts will be transformed into a more continuous signal. By this means, sensations can be made to change without any actual termination of transmission (see Chapter 2).
To summarize, it is now apparent that local anesthetic effects are more subtle, complex, and variable than has been realized in the past. This does not invalidate their use for diagnostic exercises, but does dictate caution in the interpretation of block results.
Table 38-5 Diagnostic blocks—limitations from local anesthetic systemic effects | |
---|---|
|
Systemic Effects
When assessing the response of pain perception to local anesthetic blocks, it is important to consider the systemic effect of the anesthetic as it is absorbed from the site of injection (Table 38-5). Considerable data are available to show that systemic local anesthetic analgesia reduces neuropathic pain, but does relatively little for other painful conditions.
Conventional local anesthetic blood levels that follow blockade even with large volumes of agent (e.g., 1 to 5 μg/mL after 20 mL 1% lidocaine) produce no appreciable effect on impulse conduction in normal peripheral nerves (7,8,32,50) or on cutaneous C-fiber terminal function (50). Local anesthetics also have little or no analgesic effect in animal models of acute nociception (50). Therefore, possible mechanisms for analgesia during neuropathy include effects of the drug on impulse generation in injured nerves or on processing of sensory information in the CNS.
Experimental evidence indicates that systemically administered local anesthetics affect spontaneous impulse generation arising from injured nerves. Intravenous (IV) lidocaine in subconvulsant doses produces suppression of spontaneously active nerve fibers originating from sciatic neuromas in rats (32), and impulse generation in the DRG is suppressed at even lower doses (7). Electrically evoked peripheral nerve activity remains completely intact at these doses. In addition, lidocaine decreases the sensitivity of these neurons to mechanical stimulation. Tanelian and MacIver (8) evaluated the effect of lidocaine on injury-induced discharge of corneal C- and Aδ-fibers. Concentrations ranging from 1 to 20 μg/mL suppress tonic action potential discharge, but do not block electrically evoked nerve conduction until concentrations reach 250 μg/mL.
Nerve injury is associated with significant changes in populations of Na+ channels. The tetrodotoxin (TTX)-sensitive Nav1.3 channel, which is minimally expressed in uninjured nerves except at embryologic stages of development, is upregulated in injured DRG following spinal nerve ligation (SNL) injury. Because this channel subtype recovers rapidly from inactivation, it is able to support rapid firing rates. The TTX-resistant Nav1.8 channel is upregulated in uninjured spinal nerves adjacent to the SNL injury (51). These changes are associated with the development of ectopic discharge and with hyperalgesia and allodynia. Lidocaine is nonspecific in its effect on these channels, but it is effective in blocking the activity of these upregulated channels at blood levels that do not interfere with normal nerve conduction.
Nontoxic doses of systemic local anesthetics also have depressant effects on spinal transmission of nociceptive inputs. Lidocaine significantly suppresses the spinal polysynaptic reflex evoked by stimulation of sural nerve C-fibers (50). An increase in nociceptive flexion reflex thresholds in diabetic patients, and in healthy human subjects, is observed following IV infusion of 5 mg/kg lidocaine (52).
In an effort to determine the relative potency of local anesthetics on spinal versus peripheral mechanisms, Abram and Yaksh (53) assessed the effects of IV lidocaine on hyperalgesia induced by sciatic nerve ligation and on hyperalgesia due to dorsal horn sensitization following subcutaneous formalin in rats. Intravenous lidocaine at a blood level of 1 μg/mL reversed the thermal hyperalgesia induced by nerve injury (Fig. 38-5). However, spinal sensitization was prevented only when lidocaine blood levels exceeded 6 μg/mL. This indicates that the principal effect of systemic local anesthetics on neuropathic pain is peripheral.
There have been several clinical reports of the efficacy of IV lidocaine in patients with neuropathic pain (8,52,54,55,56,57). Although some cite very transient effects (8,57), others indicate analgesic effects lasting several days or more (55,56). Doses of local anesthetic required to relieve neuropathic pain are generally 1 to 3 mg/kg. It would be unlikely, therefore, that a selective nerve root block with 3 mL 1% lidocaine would produce pain relief by a systemic effect. On the other hand, a lumbar sympathetic block, using 15 mL 1% lidocaine, might be capable of relieving neuropathic pain at a location distant from the site of injection.
Psychosocial Influences
Even though the practitioner uses diagnostic blocks to obtain specific, convincing, “hard” data, she must still be aware that the procedure is also a social interaction (Table 38-6). Although the patient may not seek to deceive the doctor, it is impossible to dissociate the experience of pain from surrounding social and psychological factors (see also Chapters 29 and 35). Important aspects to consider include the following:
Table 38-6 Diagnostic blocks—limitations from psychological issues | |
---|---|
|
Communication may be incomplete. Answers are reliable only if the physician is able to enter into the same frame of reference as the patient and to use descriptive terms in the same way (58).
The clinic is not the patient’s home (59), and unfamiliarity, stress, and anxiety may create an environment dissimilar to that in which the pain usually exists.
The patient and physician may not have the same agenda. Whereas the doctor may seek pathophysiologic information, the patient may be looking for reassurance, confirmation of suspicions or proof to persuade doubting family members, certification of disability for legal and financial reasons (see Chapter 29), or may simply wish to please the doctor (see Chapter 36).
Any of these purposes may enter into the patient’s reporting.
To diminish the ambiguities created by these psychosocial factors, a physician might choose to inject a placebo (Latin for “I please”), an inert substance with no known pharmacodynamic effect (see Chapter 36). Although desirable in many situations, the use of a placebo is an incomplete means of clarification. Problems include ethical considerations (60) of performing an invasive procedure to inject an inactive substance, and the difficulty of obtaining permission while not revealing that the injected substance is a placebo. This may be circumvented by comparing the duration of response to two local anesthetics with different pharmacokinetics (e.g., lidocaine versus bupivacaine) (61). Comparative durations of relief not in keeping with expected durations of local anesthetic effect could be interpreted as a placebo response, but this unproven method requires more subtle distinctions than the clear case of a response to saline injection, and as noted earlier, relief of pain with local anesthetic may be prolonged long beyond the drug’s presence.
A more limiting uncertainty is what to make of a patient who responds favorably to a placebo (Table 38-7). About one-third of patients obtain relief from placebos administered during acute pain (62), and they may be up to twice as likely to obtain relief of chronic pain with a placebo. For example, 82% of patients in a study of chronic arthritis reported definite improvement in pain and function after weekly subcutaneous normal saline (63). In another study, 59% had relief from a placebo tablet, whereas 57% of those who didn’t respond to a pill had relief from a subsequent placebo injection (64). In patients with causalgia, 3 mL of subcutaneous normal saline relieved spontaneous pain in 68% of patients and also relieved mechanically induced pain (allodynia) in 56% and relieved Tinel sign in 67% (65). Probability of analgesia from a placebo is proportionate to the intensity of pain (66). No personality features predict a placebo response (67). Individuals are not consistent in being responders or nonresponders, and most eventually will respond to a placebo if administered repeatedly (68). Thus, it is hard to conclude much from identifying a placebo response. Certainly, this is not a way to determine whether the pain is real.
Table 38-7 Diagnostic blocks — limitations from placebo response | |
---|---|
|
Psychologic theory explaining placebo response focuses on the subject’s expectations (69) and on conditioning (70). In the context of diagnostic blocks, the expectation of a favorable response may make analgesia more likely. By conditioning, a patient’s response to an injection is based upon what they experienced after previous similar events. It has been shown that most subjects can be trained to have a placebo response (71) and that a placebo response is more likely if the test with the active agent precedes the placebo administration (72). Other implications are that a patient’s previous analgesic experiences will condition the potency of a placebo (73) and that withdrawal of even a pharmacologically inactive therapy may lead to an increase in chronic pain (70). On a physiologic level, the placebo response is a demonstration of the descending modulation of nociception. Evidence of an opioid mechanism includes the antagonism of placebo analgesia with naloxone (74,75) and the documentation of increased cerebrospinal fluid (CSF) endogenous opioid activity after a placebo response, but this is not so if no response occurred (76). In view of this, placebos can be considered active agents in their own right (see also Chapter 36).
When local anesthetic is injected for diagnostic nerve blockade, it is very difficult to be certain that relief has not occurred through a placebo mechanism rather than through neural interruption. In general terms, placebo responses are incomplete, inconsistently repeatable, and may lack the appropriate time course for the onset or duration of the active agent. However, it is difficult to employ these generalizations in any particular clinical case. Placebo action may be as intense as the active agent, usually will mimic the active agent in dose–response and time–effect relationships (77), and may develop over as prolonged an interval as 60 minutes (Fig. 38-6) (78,79). Injections, like surgery, are especially potent placebos (77) (see also Chapter 34).
An important component of the placebo event is the practitioner. It has been demonstrated repeatedly (77,80) that even in carefully blinded protocols, unintended communication from the examiner takes place. When placebo is compared to morphine, the placebo responders have analgesia comparable to that induced by morphine, whereas if aspirin is being tested, the placebo response resembles aspirin. Patients told they might receive either a narcotic analgesic or narcotic antagonist during acute pain developed placebo analgesia (to saline) only if they were in a group that the physician knew would receive fentanyl versus saline, and not if they were in a group which the physician knew would get only naloxone versus saline. This held true even if the physician knew only which group the patient was in and not whether saline or drug was given (Fig. 38-7). The history of new therapies demonstrates the same phenomenon. Initial reports by enthusiasts are commonly contradicted by subsequent blinded and critical trials (81,82). These various observations make it clear that the physician’s convictions play a large role in generating placebo responses.
The potency and frequency of the placebo effect is underestimated by the majority of physicians and nurses (83). Far from being a minor inconvenience, this effect is a central concern in the performance of diagnostic blocks. In a sense, each diagnostic block resembles a clinical study of a drug or procedure, but with a study group of only a single subject. Convincing results are elusive without repeated testing with a blinded subject and physician.
What can be done to lessen the confusion introduced by placebo responses? Ironically, when an elaborate protocol is used to control for physician and patient bias, and adequate time is allowed for the full development of placebo response, all subjects have placebo analgesia (78). Our recommendations are to: (a) inform the patient that a placebo injection may be used at some point during diagnostic testing; (b) use more than one trial of a diagnostic block; (c) include a saline injection at least once during a series of blocks, preferably through the same needle that will then be used for the local anesthetic, allowing time between injections for questioning as to relief; (d) if possible, blind the operator performing the block as to the agent injected; (e) recognize relief from a placebo as a normal and expected event; (f) consider a response to local anesthetic more persuasive if it differs in quality, intensity, or timing from the saline injection, or if no placebo response occurred; and (g) bear in mind that relief from local anesthetic injection may still be by a placebo mechanism. A final concern is the negative placebo. From 28% (84) to 34% (85) of subjects given inactive agents will have side effects such as headache, drowsiness, asthenia, dizziness, nausea, and vomiting.
Anatomical Considerations
The use of blocks for diagnosis and prognosis depends on an assumption of anatomic consistency. We expect nerve
structures to be found in predictable places and to have predictable connections. There are important limitations to these expectations. Like any biologic feature, most anatomic parameters show variability about a norm. For example, the Tuffier line between the iliac crests crosses the vertebral column most often at the L4–L5 disc (perhaps higher on average in men than in women) (86), but the range is from as low as the L5–S1 disc to as high as the L3–L4 disc (86,87,88). A normal distribution also describes the level of termination of the spinal cord (89) and the termination of the dural sac (Fig. 38-8) (90). This indicates that surface and palpation landmarks are unreliable indicators of deep structures, which is borne out by a 50% accuracy in guessing vertebral level of needle placement without x-ray imaging (91,92,93,94,95).
structures to be found in predictable places and to have predictable connections. There are important limitations to these expectations. Like any biologic feature, most anatomic parameters show variability about a norm. For example, the Tuffier line between the iliac crests crosses the vertebral column most often at the L4–L5 disc (perhaps higher on average in men than in women) (86), but the range is from as low as the L5–S1 disc to as high as the L3–L4 disc (86,87,88). A normal distribution also describes the level of termination of the spinal cord (89) and the termination of the dural sac (Fig. 38-8) (90). This indicates that surface and palpation landmarks are unreliable indicators of deep structures, which is borne out by a 50% accuracy in guessing vertebral level of needle placement without x-ray imaging (91,92,93,94,95).
Anatomic variability is not limited to relative dimensions and positions of structures, but also includes the number and connections of anatomic items, and idealized textbook descriptions hold in only about 50% to 70% of actual subjects (96). For instance, even though patterns of vertebral segmentation are stable in the cervical and thoracic levels, lumbar and sacral regions show a marked variety of segmentation. The last lumbar or first sacral vertebra may be indeterminate in configuration, with fusion of L5 to S1 in 6.2% (sacralization of L5), or incomplete fusion of S1 to S2 in 5.3% (lumbarization of S1), and one or more sacral segments may be absent (97). The distribution of nerve roots to the intervertebral foramina is anomalous in about 8% of subjects (98,99) and includes two root pairs exiting at one level with an adjacent empty foramen (100). These variations occur because the DRG develop from a continuous sheet of neural crest tissue, and separation into segments is imprecise. The clinical consequence of aberrant arrangements is the development of anesthesia in an unexpected distribution following foraminal injection, with resulting diagnostic ambiguity (99).
Separation of somatic input into a discernible segmental pattern is a fundamental concept underlying many diagnostic blocks. No segmentation is evident upon the surface of the spinal cord or by histologic examination of its substance. It is only by the grouping of rootlets into rootlet bundles bound for a common DRG and intervertebral foramen that a pattern of segments is superimposed upon the otherwise seamless connections of the peripheral system with the CNS. There is, however, variability in the formation of segmental spinal nerves and their peripheral distribution. Multiple interconnections of adjacent rootlets and roots are found within the dural sac in all subjects, with between three and nine such intersegmental anastomoses at the upper cervical region and a similar number at the lumbosacral level (101,102). The pattern of spinal nerve contributions to the limb is highly inconsistent. For example, 28% of lumbosacral plexuses have central connections shifted proximally (“prefixed”) or distally (“postfixed”) along the vertebral column, compared to the usual pattern (103). Seven major configurations of the brachial plexus are possible, with none having more than 57% representation and with 61% of individuals differing in type between right and left (104). The resulting distribution of fibers to the skin has been mapped, using zoster eruptions, residual sensation after sectioning the roots on either side of an intact segment, absent sensation after root section or anesthesia, vasodilatation during stimulation of roots, or pain with nerve root compression and visceral disease (105). The dermatome diagrams these methods produce show considerable disagreement, especially in the extremities. Also, extensive overlap between consecutive peripheral dermatomes is evident, because the division of an individual root rarely produces an appreciable loss of sensibility. As a consequence, the sensory innervation of a particular site cannot be assigned with certainty to any segmental level, and sensory changes after local anesthetic injections about the vertebral column are variable. Segmental inconsistency is also present in the motor innervation of the extremities. Marked departure from the usual distribution of L5 and S1 motor fibers is found in 16% of subjects (106), in whom stimulation of a root produces movement typical of the other root.
Important differences in the peripheral distribution of sympathetic motor fibers are relevant to diagnostic blocks. Preganglionic axons originate only from the T1 through L2 segments. Fibers bound for tissues with cervical or low lumbar and sacral somatic innervation are deployed by the paravertebral chains. Therefore, segmental neuraxial local anesthetic application will block sympathetic innervation to tissues different from those that are somatically denervated. For instance, a low spinal anesthetic may produce intense sensory block to
the feet, ankles, calves, and buttocks (low lumbar and sacral segments) without any sympathetic changes in these areas (derived from L1 and L2). Sympathetic outflow is only weakly segmental, due to the crossing of rami communicates (107) and extensive divergence of sympathetic activity in the ganglia. Efferent sympathetic fibers supplying a cutaneous region do not necessarily arrive by the same peripheral nerve as the sensory afferents supplying that area. For example (108), the radial aspect of the dorsum of the hand receives sensory and sudomotor innervation via the radial nerve, but vasomotor innervation from the median nerve. Similarly, the lateral aspect of the foot may receive its sympathetic input from peroneal branches while transmitting sensory information in the sural nerve (109). The extent of sympathetic blockade after regional anesthetic is poorly understood and difficult to predict.
the feet, ankles, calves, and buttocks (low lumbar and sacral segments) without any sympathetic changes in these areas (derived from L1 and L2). Sympathetic outflow is only weakly segmental, due to the crossing of rami communicates (107) and extensive divergence of sympathetic activity in the ganglia. Efferent sympathetic fibers supplying a cutaneous region do not necessarily arrive by the same peripheral nerve as the sensory afferents supplying that area. For example (108), the radial aspect of the dorsum of the hand receives sensory and sudomotor innervation via the radial nerve, but vasomotor innervation from the median nerve. Similarly, the lateral aspect of the foot may receive its sympathetic input from peroneal branches while transmitting sensory information in the sural nerve (109). The extent of sympathetic blockade after regional anesthetic is poorly understood and difficult to predict.
Anatomic variability is also evident in the distribution of injected solutions. Spread is guided by the vagaries of tissue pressures and adherence, and it may be entirely unidirectional, rather than expanding concentrically from the injection site. The vertebral canal is a common pathway for aberrant injectate flow after injections near the vertebral column. As pressure of the solution rises at the injection site, spread to the canal may occur, because pressure in the canal does not rise above CSF pressure (about 15 cm H2O), since inflow displaces CSF. Epidural anesthetic effect may be an undesired component of various diagnostic blocks (facet, lumbar sympathetic, stellate, nerve root, and plexus blocks).
Less is known about the patterns of visceral sensory connections. Visceral receptive fields are large and overlapping, and extensive convergence of afferent traffic is evident at many CNS levels (24). Most visceral pain travels in sympathetic nerves, but afferents from the thoracic organs, the pancreas, and biliary tree ascend in the phrenic nerve and pass to the thoracic cord via medial branches of the sympathetic chain. From the sigmoid colon, rectum, neck of the bladder, prostate, and cervix of the uterus, most visceral afferent fibers retrace the route of parasympathetic efferent neurons, entering the cord in the posterior roots of S2–S4. A few fibers from these organs ascend in the prevertebral plexuses to enter at L1–L2. Pain that is not relieved by blocks of sympathetic pathways may still be visceral in origin but transmitted by these nonsympathetic routes (see Chapter 31, Figs. 31-20,31-21,31-22).
The role and even presence of nociceptive fibers from the limbs that travel in sympathetic structures has been debated (110,111). Lumbar sympathetic block prevents the poorly localized dull ache during surgical manipulation of the femoral vein or from lower extremity thrombophlebitis (112,113). Surgical sympathectomy blocks responses to venous distension in canine lower extremities (114) and almost eliminates the aching and stinging pain from cold exposure of human extremities (115). Sympathetic block also interrupts pain from mechanical stimulation of the femoral medullary cavity (116). These observations indicate that interruption of afferent sensory impulses travel from vascular structures in the extremities via sympathetic pathways which, when blocked by diagnostic sympathetic blockade, could be falsely interpreted as an indication of an efferent sympathetic pathogenesis of pain. One recent analysis attributes most “sympathetic dependent” pain to visceral afferent activity (117) (see also Chapter 39 and Chapter 31, Fig. 31-8).
Deep somatic pain from bones, joints, muscles, and fascia shares many features of visceral pain including poor somatotopic localization, referral of pain to distant sites, and a particular ability to generally increase CNS excitability, manifest as elevated motor and autonomic reflexes (110). Additionally, the fibers from many deep somatic elements (costovertebral joint, posterior and anterior longitudinal ligaments, anular ligament of the intervertebral disc, dura) traverse the sympathetic rami and chain (118,119,120,121). It is likely that some pains relieved by sympathetic blocks are unrelated to sympathetic efferent activity (sympathetically maintained pain) and instead are deep somatic pains transmitted by sympathetic pathways (117).
Methodology
Diagnostic blocks should be performed in a manner that yields the most certain information possible, in order not to add to the inherent ambiguity of clinical pain. In many cases, needle position should be confirmed by radiologic imaging. Determination of the segmental level is unreliable without confirmation by radiography, and studies of a variety of injection procedures have shown inadequate consistency of needle placement without imaging (91,122,123,124). Since small volumes of local anesthetic need to be used to minimize spread to undesired nerves, meticulous needle placement is required to assure adequate blockade of the desired nerve. Injection of a small amount of radiopaque contrast, prior to the anesthetic, can identify passage into an unwanted space (vascular, subarachnoid) or in an ineffective direction. The usefulness of guiding needle insertion by the nature of provoked pain is limited by the lack of specificity of deep sensations (125,126).
Pain before and after blockade should be evaluated by asking the patient to rate the intensity, between 0 (none) and 10 (worst imaginable), to facilitate communication and documentation. Provocative measures, such as palpation of a tender area or joint movement, may help the patient and clinician discern changes in incident pain, when compared before and after the block. For instance, in a patient who has pain only when ambulating, implantation of a pump for intrathecal opioid treatment is not supported by a prognostic trial during which the patient is kept in bed. Not only may a patient with spontaneous pain respond differently than if he has induced pain, but blocks used may act differently upon pain induced by repetitive dynamic mechanical stimulation (dynamic mechanical allodynia) versus steady pressure (static mechanical allodynia) (127). Careful examination is required to discern these subtle block effects. If the patient is not having the usual pain at the time of a diagnostic block, little can be learned about the pain’s mechanism. When pain is relieved by neural blockade, the duration of analgesia should be determined. Relief lasting only the expected duration of the anesthetic effect suggests an ongoing peripheral focus of nociception. If relief obviously outlasts the anesthetic, a central potentiating process may be involved.
Pain relief, however, cannot be the only measured parameter. Direct confirmation of actual neural blockade is necessary, because performing the procedure correctly and with care does not guarantee that the intended nerve will be anesthetized and the desired physiologic change achieved. Detailed sensory and motor testing before and after injection can identify somatic nerve block effects. Meticulous care may be necessary to delineate sensory changes. For instance, vibration transmitted through the skin and soft tissue may stimulate receptors at a considerable distance, creating the misimpression that an area is incompletely insensate (128). Tactile threshold changes can be identified by testing with fibers of different stiffness (von Frey hairs), and fast pain (Aδ) sensation can be examined by a scratch, for example using the folded corner of a foil alcohol pad wrapper or a broken tongue blade.
More elaborate methods are necessary for an adequate test of C-fiber (slow pain) sensation. Stimuli should be performed as consistently as possible since a stimulus that is more intense, more frequently repeated, or more broadly distributed may be perceived, whereas weaker stimuli are blocked (129).
Inconsistent stimulation may cause confusion. For instance, subtle somatic blockade may be missed during diagnostic sympathetic block if application of stimuli is more vigorous after the block than during baseline examination.
Inconsistent stimulation may cause confusion. For instance, subtle somatic blockade may be missed during diagnostic sympathetic block if application of stimuli is more vigorous after the block than during baseline examination.
Many measures have been used to judge the efficacy of sympathetic blockade, although none has become an accepted standard. Horner syndrome documents only blockade of sympathetic fibers to the head. Skin resistance response (sympathogalvanic response) (130,131) and pulse amplitude changes (132,133) are difficult to quantify. Microneurography (131) is invasive and requires elaborate equipment and expertise, as does laser skin blood flow measurement (134). Sweat testing (135) is cumbersome, time-consuming, and not well accepted by patients, and therefore not widely used. Most common is the measurement of skin temperature by thermography or contact thermometry. A temperature increase of 1.0°C to 3.0°C is typically used (136,137,138) as a threshold for confirming the onset of sympathetic blockade, but the method is ineffective if skin is warm at the outset of a block. Although local anesthetic blockade of sympathetic activity to the extremities produces vasodilatation, vasoconstriction follows segmental block of sympathetic fibers to the trunk (37), possibly by blockade of sympathetic vasodilator fibers (139). Skin temperature in pathologic conditions is controlled by a balance between sympathetic vasoconstriction and vasodilatation from release of vasoactive peptides from C nociceptors during antidromic activity (140). Temperature change in the field of a blocked peripheral nerve will depend on the relative contribution of these opposing systems.
Pain caused by the procedure may result in a confused diagnosis, because relief following the injection may be due to the termination of the iatrogenic pain and not of the preexisting pain for which the block was done. Also, intense pain from the procedure may diminish the perceived severity of the original pain (noxious counterirritation) (141), creating the illusion that neural blockade effects relieved the pain. For these reasons, the diagnostic blocks should be performed using measures to limit discomfort. Judicious use of local anesthetics in the superficial tissues, small needles, and careful needle guidance limit the pain of the procedure.
Specific Procedures
In the format adopted below, commonly used diagnostic blocks will be considered individually, first with regard to the rationale behind the procedure, including indications for the block. The discussion of technique that follows also considers methods for monitoring response to the block and for identifying possible complications. (The techniques of blocks discussed more completely elsewhere in this book are covered briefly.) Limitations are reviewed, focusing on sources of error in interpreting results from the injections. If studies of the diagnostic utility of the procedure are available, these are reviewed with emphasis on documentation of success and measures of the diagnostic value. Finally, an evaluation of the utility of the block is offered.
Clinical studies of the blocks vary in quality. Important considerations include entrance criteria (type of patients or normal subjects studied), study size, and the use of controls. The prevalence of placebo responses in pain patients greatly weakens the relevance of studies in which no controls or blinding was used. Where possible, neural blockade tests are evaluated numerically, using standard definitions (see the table immediately following) (142). The importance of the false-positive rate (how often patients without a condition will nonetheless have a positive test) and false-negative rate (how often a patient with disease will have a negative test for it) is evident, because the rates vary inversely with specificity and sensitivity respectively. (Sensitivity = 1 – false negative rate; specificity = 1 – false positive rate.) For many painful conditions, however, a credible standard to document the disease for comparison with test results is unavailable, such as when sympathetic involvement is suspected or with cervicogenic headache. At worst, the block under scrutiny may be the defining gold standard. For these blocks, numerical values for diagnostic efficacy are elusive. In many studies, ethical reasons limit operative confirmation of disease to only those patients with positive results from the diagnostic block. The false-negative rate is therefore unknown, and the false-positive rate, which requires knowledge of true disease incidence in the entire group, also cannot be calculated. From these studies, only the positive predictive value (frequency of confirming disease in those with a positive test) can be derived.
Disease Present | Disease Absent | |
Test Positive | a | c |
Test Negative | b | d |
Sensitivity (true positive rate) | a/(a + b) | |
False-positive Rate | c/(c + d) | |
Specificity (true negative rate) | d/(c + d) | |
False-negative Rate | b/(a + b) | |
Positive Predictive Value | a/(a + c) | |
Negative Predictive Value | d/(b + d) |
The proper interpretation of a positive test must take into consideration the prevalence of the condition. For example, a test with 95% sensitivity will have a positive result in 5% of healthy subjects. If the condition being sought is rare (if, for instance, it occurs in only 2% of the test group), false-positive responses will outnumber true positive tests, and the majority of positive results will occur in subjects who actually are healthy. This issue is especially relevant to the study of painful conditions by diagnostic blocks, since the incidence of most of these maladies is low or unknown.
Tissue Infiltration
Rationale
If nociceptive impulses are thought to arise from a particular site (for example, from a scar, painful structure in a specific muscle, inflamed joint, bursa, or tendon sheath), then the injection of local anesthetic into that site should be of help in establishing the diagnosis. Failure to relieve tenderness by infiltration of superficial tissues, like skin or muscle, focuses attention on a deeper site (bone, joint, nerve root). Painful scars generally are thought to be caused by the development of small neuromas. Although some scars are diffusely tender, most scar pain is associated with very localized areas of tenderness. Some patients who experience relief from local anesthetic will have lasting relief if depot steroids are injected subsequently. When inflammatory processes are the cause of the pain, local anesthetic infiltration may be of prognostic benefit in predicting the response to subsequent steroid injections.
Technique
A skin wheal followed by injection of a small amount of saline in the vicinity of the planned procedure may indicate whether
analgesia obtained from local anesthetic injection is due to placebo response. A small amount of local anesthetic is then injected into the affected muscle, joint, tendon sheath, scar, bursa, or like site. It is helpful to determine whether needle placement and local anesthetic injection (as well as the placebo injection) reproduce the clinical pain, and whether local anesthetic injection relieves the pain at rest as well as the pain produced by maneuvers that usually aggravate the pain.
analgesia obtained from local anesthetic injection is due to placebo response. A small amount of local anesthetic is then injected into the affected muscle, joint, tendon sheath, scar, bursa, or like site. It is helpful to determine whether needle placement and local anesthetic injection (as well as the placebo injection) reproduce the clinical pain, and whether local anesthetic injection relieves the pain at rest as well as the pain produced by maneuvers that usually aggravate the pain.
Local infiltration techniques are relatively benign in terms of potential for adverse effects. Infection is always a potential problem in immunocompromised patients, and bleeding may be troublesome if coagulation function is impaired. Extensive infiltration of painful muscles conceivably could result in local anesthetic toxicity or muscle damage (143). In general, the occurrence of complications relates to proximity of the injection to other structures. Injection of the rhomboids or trapezius muscles could cause pneumothorax. Infiltration of muscles or scars near the neuraxis may allow spread of drug epidurally or intrathecally. Infiltration of occipital scars overlying bony cranial defects, following posterior fossa surgery, could mean that drug might be injected into the intracranial CSF, or even into the brain itself. Careful monitoring of blood pressure should be carried out when neuraxial or intracranial injections are possible.
Trigger-Point Injection
Rationale
Myofascial pain syndrome is characterized by pain arising from affected muscles, pain associated with movement of those muscles, and reproduction of pain with palpation of well localized “trigger points” in the affected muscle (144). By definition, stimulation or palpation of trigger points causes referred pain, perceived some distance from the site of palpation. Usually, the involved muscle is felt as a tight palpable band. Myofascial syndrome often is found in association with other painful disorders, such as facet arthropathy or radiculopathy, and it is often helpful to determine whether a patient’s pain is predominantly myofascial, because appropriate treatment may be very different if such is the case. Other means of documenting myofascial pain, such as electromyelography (EMG), have not proved reliable (145,146), although elevated endplate noise recorded during EMG has recently been proposed as a marker of myofascial trigger points (147). Muscle tenderness also is seen in fibromyalgia, which differs from myofascial pain syndrome in that tender points in the muscle are much more diffuse and numerous, and usually symmetrical, and palpation generally produces local, but not referred pain (148). Trigger-point injections, particularly if repeated several times, may have therapeutic benefit for myofascial pain syndrome but not for fibromyalgia (149).
Technique
The area of maximum tenderness is identified within the affected muscle. It may be helpful to immobilize the muscle between the thumb and forefinger. After antiseptic preparation of the skin, a small-gauge needle is introduced into the trigger point. Often a brief twitch of the affected muscle is noted. The patient should be questioned about the location and intensity of the pain evoked by needle placement. Exacerbation of the pain and the presence of referred pain at this time helps confirm the diagnosis of myofascial pain syndrome. Following the injection of several milliliters of local anesthetic, the degree of pain relief and the presence or absence of tenderness is reassessed. Pressure algometry may be used to determine the degree of tenderness before and after injection.
A variety of local anesthetics have been used for trigger-point injection. From a diagnostic point of view, there is little reason to choose one over another. Ropivacaine may produce less pain when injected (150). Some clinicians choose to avoid bupivacaine, because accidental blockade of nearby neural structures results in prolonged effects, toxic local anesthetic reactions are potentially more serious, and bupivacaine produces more muscle degeneration than do other anesthetics (151). Ironically, the predictable and selective destruction of mature myocytes by local anesthetic infiltration (143) might be the therapeutic mechanism of long-term response to trigger-point injection, because it encourages the growth of a new generation of myocytes, in which case bupivacaine is a suitable agent. Reproduction of pain during injection and relief of pain after injection suggest that myofascial pain is at least partially responsible for the patient’s pain.
The small volume of anesthetic used in trigger-point injection makes this a safe procedure. However, toxicity may result if multiple blocks are performed or if even a small dose reaches the subarachnoid space (152).
Clinical Studies
No studies conclusively demonstrate that trigger-point injection can distinguish myofascial pain from other etiologies, since there is no independent gold standard for identifying myofascial pain other than clinical features. However, utility of this procedure has been shown in studies in which the contribution of myofascial pain is suggested through injection. For instance, pain may be relieved by trigger-point injection in a subset of patients with postthoracotomy pain syndrome (153) or pain following radical neck surgery (154). Myofascial pain presenting as toothache has similarly been identified (155).
Limitations
Pain relief after trigger-point injection does not guarantee that myofascial pain is the principle cause of pain. Placebo effect and systemic uptake of local anesthetic, particularly after multiple injections, may be the cause of the improvement, as may be spread to adjacent nerves. For instance, injection of the piriformis muscle is likely to have some effect on the sciatic nerve, which either penetrates or passes in contact with the muscle. Some doubt about the specificity of the technique is raised by reports showing comparable efficacy from less specific techniques, such as dry needling of trigger points (156) (see also Chapter 34) and jet injection of local anesthetic into the skin overlying trigger points (157).
Evaluation
Reproduction of pain during injection followed by relief of pain is helpful in confirming the tissue site (scar, muscle, etc.) as the focus of nociceptive activity, if the duration of relief is at least as long as the expected duration of local anesthetic. Controlled studies have not confirmed this belief.
Somatic Nerve Block
Rationale
A common reason to perform diagnostic peripheral nerve blocks is to determine the likelihood of success after surgical decompression or neurolysis of a peripheral nerve. Diagnostic blocks may be performed before a planned peripheral nerve section, neurolytic block, or cryoanalgesia lesion. Entrapment neuropathies include digital nerve entrapment (Morton neuroma), carpal tunnel entrapment of the median nerve, and tarsal tunnel entrapment of the tibial nerve. Posttraumatic neuropathy of the ilioinguinal and iliohypogastric nerves can occur following herniorrhaphy.
Technique
Techniques for specific peripheral nerve blocks are covered in Chapters 13, 14, and 16,17,18,19. When performing diagnostic blocks, it is important to use small volumes of anesthetic solution, to document that appropriate sensory blockade is achieved, and to ensure that the block is limited to the intended nerve distribution. In assessing relief of pain, one should determine the duration of the pain relief and the duration of sensory block. If pain returns long before the return of sensory function, the analgesia may be a placebo response. Use of bupivacaine for peripheral diagnostic blocks may be preferable to the use of shorter-acting agents, because it provides a long interval during which placebo responses may subside and a prolonged period for patients to experience the effect of the block. In general, diagnostic blocks are performed with relatively small volumes of local anesthetic (usually 2–3 mL for small nerves, 5 mL for medium-sized nerves, e.g., median nerve at the wrist, and 10 mL for large nerves), and the risk of toxic reactions is small. Intercostal blocks done near the spinal column may be associated with spread of drug to the subarachnoid space, if the needle is placed within the perineurium.
Limitations
It must be kept in mind that relief of pain following blockade of the appropriate nerve does not necessarily confirm the diagnosis of neuropathy at that site. There may be a nociceptive source of pain within the distribution of the blocked nerve, or there may be a neuropathic source of pain proximal to the site of block (e.g., radiculopathy or plexopathy) that may be relieved by the procedure (11,12,13).
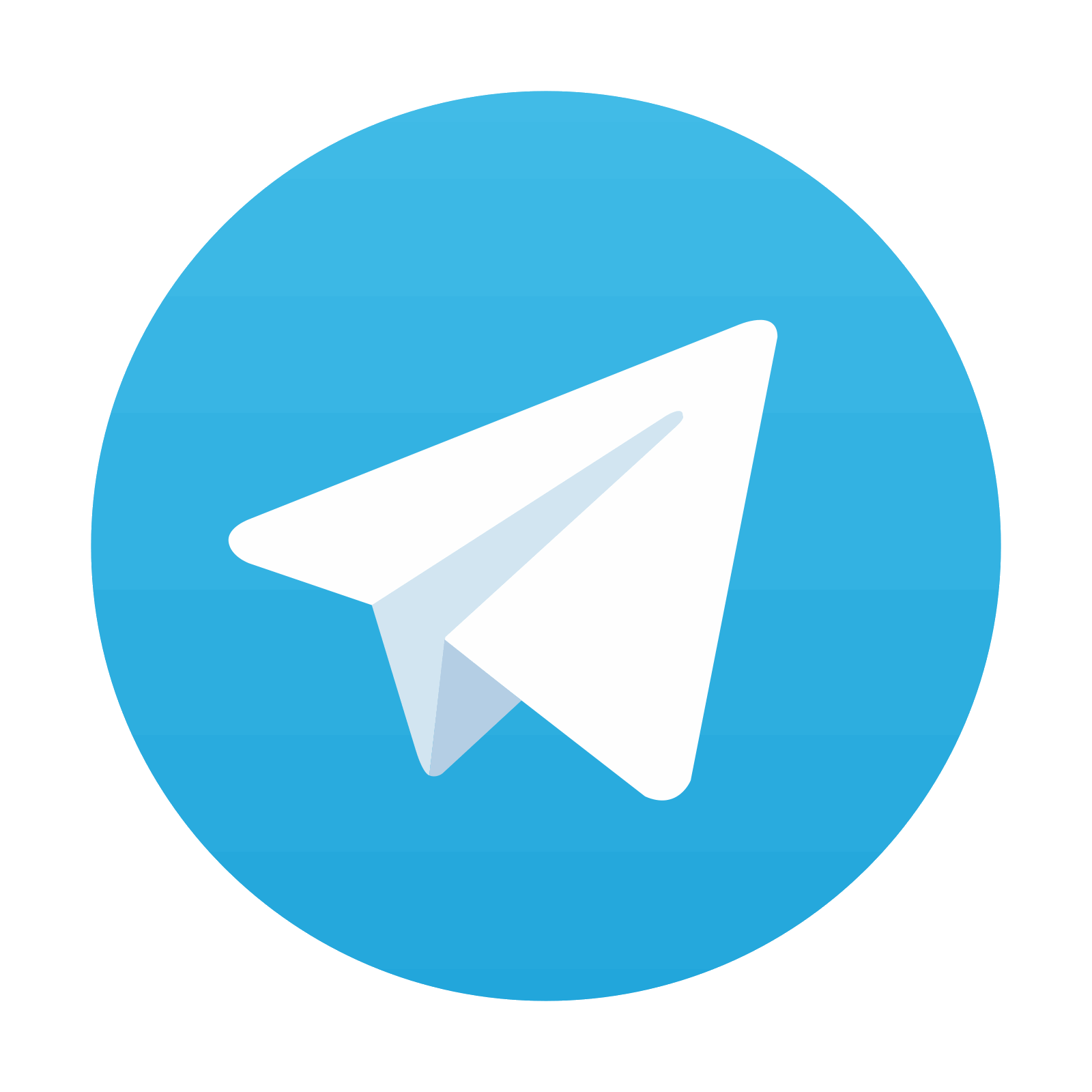
Stay updated, free articles. Join our Telegram channel
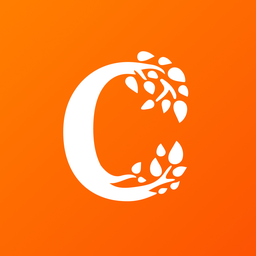
Full access? Get Clinical Tree
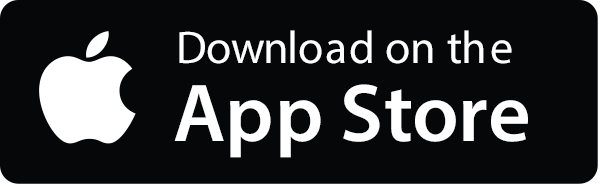
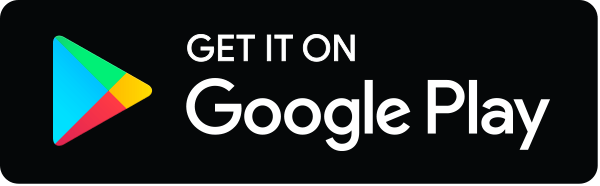