Bone Marrow and Blood Cell Collection
Cells used for transplants are most often collected from the blood, but may also be collected from the bone marrow or umbilical cord blood (22–27). Collection of blood cells is an outpatient procedure accomplished with an apheresis device, such as the Cobe Spectra. In the context of autotransplants, recipients often receive chemotherapy and/or hematopoietic growth factors to increase numbers of circulating blood cells and therefore blood cells collected. Normal allotransplant donors often receive only hematopoietic growth factors. The timing of apheresis correlates with the method used to increase numbers of cells collected: apheresis is usually done for 1 to 3 days, starting 4 days after beginning hematopoietic growth factor therapy. In contrast, when chemotherapy is used, apheresis is usually done for 1 to 3 days when neutrophils are present at a level of greater than or equal to 1 × 109 cells/L; this is usually 10 to 16 days after induction of chemotherapy. The advantages of collecting blood rather than bone marrow cells include: no need for anesthesia; use in subjects with hypocellular, fibrotic, or cancer-infiltrated bone marrow; and a more rapid posttransplant bone marrow recovery. A disadvantage of using blood as opposed to bone marrow cells is an increased incidence and severity of chronic GvHD, likely because blood contains 10-fold more T cells, the cells causing chronic GvHD (26). The cost of blood cell collection is similar to collecting bone marrow cells. Complications of blood cell collection are rare, but include infection, anaphylaxis, and hypocalcemia.
Bone marrow cells are collected in the operating room under general, epidural, spinal, or caudal anesthesia. The donor may be admitted on the day of the procedure and discharged the next day. Sometimes, an autologous unit of red blood cells (RBCs) unit is collected 3 to 4 weeks before the procedure for autotransfusion. Complications of bone marrow collection are rare but include the risks of anesthesia: hypotension, nausea, vomiting, cardiac arrest, pulmonary emboli, pain, hemorrhage, and infection.
Cord blood cells are obtained from the umbilical cord and placental blood at the time of birth. The target is to collect 2 to 4 × 107 nucleated cells/kg recipient weight. This is more than 10-fold less than the 2 to 4 × 108 nucleated cells/kg recipient weight collected from the bone marrow. Cord blood cell transplants are often restricted to children because of the small number of cells collected. The potential advantages of cord blood are a higher proportion of progenitor cells compared to bone marrow and possibly less GvHD from fewer T cells. Recently, adults have been transplanted with double umbilical cord blood cells with some success (28).
Bone Marrow and Blood Cell Infusion
Bone marrow and blood cells may be frozen in dimethyl sulfoxide (DMSO) for later use (29,30). The intracellular contents of cells destroyed in the freezing and thawing processes—and DMSO itself—may cause hypotension, anaphylaxis, or dysrhythmias, including transient heart block (31). To avoid complications, subjects are premedicated with diphenhydramine hydrochloride (Benadryl) and methylprednisolone sodium succinate (Solu-Medrol). Intubation equipment and epinephrine should be available at the bedside when cells are infused. If hypotension occurs, the infusion is slowed or temporarily interrupted until the blood pressure stabilizes. If the bone marrow or blood cells have not been frozen, the risk of anaphylaxis is similar to a standard blood transfusion, and premedication is unnecessary.
Bone marrow and blood cell collections are routinely analyzed for quality control at various times during collection, processing, storage, and infusion. Approximately 1.2% of cultures obtained during these processes are found to contain bacteria (32). Most cultures show coagulase-negative Staphylococcus sp., which colonize the skin; pathogenic gram-negative bacteria are occasionally present. Bone marrow and blood cell collections inconvenience the donor and cost approximately $16,000. Thus, despite positive culture results, most centers infuse the cells after appropriate antibiotic coverage; although controversial, this approach has generally been without adverse effects.
Fluids and Hypotension
High-dose chemotherapy and radiation damage vascular endothelial cells, resulting in extravascular leakage of fluids. Furthermore, GvHD and cytokines such as tumor necrosis factor (TNF), interleukin 2 (IL-2), and interferon-gamma (IFN-γ) contribute to a posttransplant capillary leak syndrome (33–36). In addition, subjects often receive large volumes of intravenous (IV) fluids from drug infusions, parenteral nutrition, and prophylaxis for hemorrhagic cystitis. Consequently, all transplant recipients gain weight, with the result that diuretics are frequently given to maintain baseline weight and mitigate fluid retention. If hypotension develops, emphasis should be placed on early invasive cardiovascular monitoring, inotropic support, and irradiated packed RBC transfusion to maintain intravascular oncotic pressure. Aggressive hydration may precipitate pulmonary and peripheral edema, even with normal pulmonary artery wedge pressure and right atrial pressure.
Electrolyte Balance
Electrolyte abnormalities are common in transplant recipients, resulting from the underlying disease, prophylactic hydration for hemorrhagic cystitis, diarrhea, parenteral nutrition, renal insufficiency, diuretics, and other medications. Ifosfamide, especially when combined with carboplatin, causes a Fanconi syndrome–like renal tubular acidosis 3 to 7 days after the pretransplant conditioning regimen (37,38). The resulting normal anion gap acidosis may be treated with sodium bicarbonate. Other drugs associated with renal tubular wasting of electrolytes are amphotericin, foscarnet, and aminoglycosides. The syndrome of inappropriate secretion of antidiuretic hormone (SIADH) may result from high-dose cyclophosphamide and/or ifosfamide. Cyclosporine may cause hypomagnesemia and hypokalemia or hyperkalemia; hypomagnesemia increases the risk of cyclosporine-associated seizures. Tumor lysis syndrome is rare, as most transplant recipients have relatively few cancer cells and receive intensive hydration. Finally, uric acid, a major blood antioxidant, is markedly decreased soon after a transplant, independent of allopurinol, which is often given (39).
Blood Product Transfusions
Subjects receiving transplants are immune compromised and at risk for transfusion-associated GvHD. All cellular blood products contain white blood cells (WBCs), including immune-competent T cells, and should be irradiated to 25 Gy (40).
Cytomegalovirus (CMV) infection is another risk. Allotransplant recipients should receive CMV-negative blood product transfusions, especially when the recipient is CMV seronegative (41–44). If CMV seronegative blood is unavailable, removal of contaminating WBC with an inline microfilter is an alternative (45,46). When an allotransplant recipient is CMV seropositive, no special CMV-related precautions are needed. Because autotransplant recipients do not develop GvHD or receive posttransplant immune suppression, the risk of CMV-related infection is low (47), and no special CMV-related precautions are needed.
TABLE 76.2 Donor–Recipient ABO Incompatibility | ||
![]() |
Special consideration is needed regarding ABO-compatibility between recipient and donor (48–50). As engraftment occurs, there is a gradual switch of RBCs to the donor ABO type with a transition period when RBCs with recipient and donor ABO types are present in the blood. When there is A and/or B incompatibility between recipient and donor, there is the possibility that residual anti-A or -B recipient antibodies may react against donor RBCs. Also, B cells in the graft may produce anti-A or -B antibodies against residual recipient RBCs. This complexity of blood product transfusion support should be viewed in terms of whether there is major or minor ABO incompatibility between the recipient and the donor (Tables 76.2 and 76.3). A major ABO incompatibility occurs when the recipient has antibodies to the donor RBC phenotype, for example, recipient group O, donor group A. To prevent immediate RBC destruction by pre-existing recipient antibodies, RBCs should be removed from the graft. Posttransplant, the recipient should receive recipient ABO-type or O-type RBC transfusions from which plasma and platelets are removed. With a minor ABO incompatibility, the donor has anti-A and/or anti-B antibodies to the recipient’s RBC ABO type, for example, donor O type, and recipient A or B type. Donor anti-A and/or anti-B antibodies should be removed from the graft. Posttransplant, the recipient should receive O-type RBC transfusions and recipient ABO-type plasma and platelets. When recipient and donor have anti-A and/or anti-B antibodies to each other’s ABO type, for example, recipient A type and donor B type, there is a combined major/minor ABO incompatibility. In this instance, RBC and plasma should be removed from the graft. Posttransplant, the recipient should receive O-type RBC transfusions and AB-type platelets and plasma.
TABLE 76.3 ABO Type of Blood Components Used in Patients Who Have Received an ABO-Incompatible Transplant |
![]() |
Despite using ABO-compatible platelets, many subjects fail to respond to platelet transfusions early posttransplant. Causes include fever, hepatic sinusoidal obstruction syndrome/veno-occlusive disease (SOS/VOD), drugs, infection, disseminated intravascular coagulation (DIC), and microangiopathic hemolytic anemia related to cyclosporine and/or GvHD (51).
Infection Prevention
Tactics to prevent bacterial, viral, and fungal infections vary considerably between centers (52–54), reflecting the absence of definitive studies and frequent availability of new drugs. Types of infections in transplant recipients correlate with the interval posttransplant. Tactics to prevent bacterial infections early posttransplant are based on two considerations: most infections arise from endogenous microorganisms; and in studies of neutropenic animals, the oral inoculum of gram-negative bacteria required to cause death is increased by colonization of the gastrointestinal tract with anaerobic bacteria. This has led to selective aerobic gastrointestinal decontamination, first, with nonabsorbable antibiotics such as gentamicin, vancomycin, and nystatin, and later, with absorbable antimicrobials selective for aerobes such as oral quinolones (55–57).
Standards for prevention of infection vary from complete isolation in laminar airflow (LAF) rooms to none. In LAF rooms, the subject is in a sterile environment; persons entering must be gloved and gowned, and food is sterilized or has a low microbial content because it is autoclaved or microwaved (58–61). Prophylactic oral antibiotics are given to destroy enteric pathogens, which not only are reservoirs for infection, but also may function as super-antigens that increase the severity of GvHD (62). The minimal standards to prevent bacterial infections include the following:
- A transplant unit set aside from general hospital, patients, and visitor traffic.
- High-efficiency particulate air (HEPA) filtration to prevent iatrogenic Aspergillus sp. infection (63,64).
- Careful handwashing before entering a patient’s room.
- A diet without fresh salads, vegetables, or fruits, as these may be contaminated with gram-negative bacteria, or without pepper, as this may be contaminated with an Aspergillus spp (65).
Other measures, such as shoe covers, gloves, masks, and gowns and low microbial diets and anterooms are also commonly used but their effectiveness is debatable. Bacterial prophylactic measures are generally discontinued when granulocytes exceed 0.5 × 109 cells/L.
Tactics to prevent fungal infections include the use of oral triazoles, such as itraconazole, voriconazole, posaconazole, or fluconazole, given orally or intravenously for the first month posttransplant (66). The azole antifungals are effective against most Candida spp; in transplant recipients, fluconazole is ineffective and itraconazole, voriconazole, and posaconazole are more effective against Aspergillus spp. Most Aspergillus spp infections are iatrogenic and preventable by HEPA filtration of rooms. Subjects with prior aspergillus infection are at high risk of recurrence (67), especially when there is:
- Prolonged postransplant neutropenia
- Advanced cancer
- A brief interval from beginning systemic antifungal therapy to the transplant
- Severe acute GvHD
Persons with prior aspergillosis should receive amphotericin, voriconazole, posaconazole, or caspofungin early posttransplant (68).
Herpes simplex reactivation is usually prevented by using intravenous or oral aciclovir for the first month posttransplant (69,70). Treatment thereafter results in frequent aciclovir resistance and delays the development of natural immunity.
Fever and Neutropenia
Transplant recipients are immune compromised because of neutropenia, breakdown in mucosal barriers (e.g., mucositis), invasive therapies (e.g., indwelling urinary bladder [IUBC, Foley] catheter or central venous lines), immune suppressive drugs (e.g., cyclosporine, corticosteroids, and methotrexate), and GvHD. Lymphocyte function is also affected by thymic involution, weak proliferative responses to T- or B-cell mitogens, and inverted CD4+/CD8+ ratios for 6 months after autotransplants and more than 1 year after allotransplants (71, 72). When an allotransplant is complicated by chronic GvHD, normal lymphocyte function may never return (73). The risk of infection depends on the genetic link between the donor and the recipient, graft type, and posttransplant immune suppression.
In transplant recipients with fever—temperature greater than or equal to 38°C—and granulocytes less than 0.5 × 109 cells/L (74), one should try to identify a possible infection source using a chest radiograph, blood, and urine cultures and physical examination with emphasis on intravenous catheter sites, the perineal and, oropharyngeal regions and the sinuses. Often no source is identified and broad-spectrum antibiotics are begun. The choice of antibiotics may include an antipseudomonal penicillin, aminoglycoside and vancomycin, or a third-generation antipseudomonal cephalosporin or carbapenem. Transplant recipients commonly receive loop diuretics such as furosemide, which can additively increase ototoxicity. Allotransplant recipients receive cyclosporine, whose nephrotoxicity is increased by aminoglycosides. Recurrent or persistent fever for 3 to 5 days without source in a person with granulocytes less than 0.5 × 109 cells/L is an indication for empiric antifungal therapy with amphotericin. The renal toxicity of amphotericin is enhanced by cyclosporine and aminoglycosides.
Mucositis
The severity of mucositis depends on the components of the preconditioning regimen. The non–bone marrow dose-limiting toxicity of etoposide, busulfan, cytarabine, thiotepa, and paclitaxel is mucositis; radiation also contributes to mucositis. Not surprisingly, conditioning regimens containing these drugs and/or radiation are associated with severe mucositis. Other risk factors include posttransplant methotrexate and pretransplant IFN-γ. Methotrexate should be withheld if severe mucositis develops, whereas IFN-γ should be discontinued at least 2 to 4 weeks before giving radiation.
Management of mucositis includes good oral hygiene, using, for example, saline, chlorhexidine, and nystatin rinses, and topical analgesics (75,76). Opioids are often needed, and should be given intravenously on a schedule—as opposed to PRN (as needed)—or using patient-controlled analgesia (PCA). Severe mucositis may require prophylactic intubation for airway protection. Ultimately, the resolution of mucositis generally correlates with recovery of blood granulocytes. New drugs, such as palifermin (recombinant human keratinocyte growth factor-1) reduce the incidence and severity of oral mucositis (77).
Diarrhea
Diarrhea in transplant recipients may be caused by high-dose drugs and radiation, antibiotics, bacterial and/or viral infections, GvHD, and other factors (78–80). The pretransplant conditioning regimen is the most common cause of diarrhea within 2 to 3 weeks posttransplant. Nevertheless, an infectious cause should always be considered, including Clostridium difficile and Escherichia coli (0157:H7), CMV, herpes simplex, adenoviruses, rotaviruses, echoviruses, astroviruses, Norwalk virus, Coxsackie virus, Strongyloides spp, Giardia spp, and Cryptosporidium spp. GvHD also causes diarrhea; the diagnosis can be confirmed by intestinal biopsy showing loss of crypts, vacuolization of crypt epithelium, karyorrhectic apoptotic debris, microabscesses, and, in severe cases, ulceration and denudation of the epithelium. Therapy is directed toward appropriate antibiotics for infections and immune suppression for GvHD. Conditioning regimen- and GvHD-associated diarrhea may respond to octreotide, a somatostatin analog whose mechanism of action is partly through the inhibition of secretory hormones (81). Some viral infections, such as CMV, respond to ganciclovir, foscarnet, or cidofovir (82).
Hemorrhagic Cystitis
Hemorrhagic cystitis, occurring 2 to 3 weeks posttransplant, usually results from drugs in the pretransplant conditioning regimen, such as cyclophosphamide, ifosfamide, or etoposide (83–85). Prophylaxis for hemorrhagic cystitis includes hydration and diuretics to maintain urine output at 2 mL/Kg per hour (86,87). Sodium mercaptoethane sulfate (Mesna) is often used, especially with high-dose cyclophosphamide or ifosfamide (88). Mesna is inert in plasma but is hydrolyzed in the urine to reactive monomers that conjugate alkylating drugs. It has a short half-life and is therefore given by continuous intravenous infusion. Complications of hemorrhagic cystitis are uncontrolled bleeding and clotting of the ureters or urethra, resulting in acute kidney failure. Obstruction of the ureters by clots may be asymptomatic or cause kidney colic from ureteral spasm. Severe pain may occur in the back or flank and radiate into the groin or genitals; occasionally it is necessary to insert ureteral stents or a ureterostomy.
Therapy of hemorrhagic cystitis consists of using a Foley catheter to irrigate the bladder with normal saline at 250 mL/hr to prevent intravesicular clotting. Platelets should be maintained at more than 50 × 109 cells/L with platelet transfusions and RBC transfusion should be given to replace blood loss. Discomfort from local bladder spasm can be treated with antispasmodic agents such as oxybutynin chloride (Ditropan). In severe cases, arterial embolization or cystectomy may be necessary.
Hemorrhagic cystitis seen more than 2 weeks posttransplant can result from pretransplant conditioning or viral infection due to, for example, adenovirus, CMV, JC, or BK viruses of the bladder epithelium (89–91); except for CMV, there are no effective antiviral drugs.
Sinusoidal Obstruction Syndrome/Veno-Occlusive Disease of the Liver
SOS/VOD of the liver is caused by drugs and/or radiation in the pretransplant conditioning regimen within 1 to 3 months posttransplant (92). Unlike the Budd–Chiari syndrome with thrombosis of the large hepatic veins, SOS/VOD arises from thrombosis of the central venule. High-dose therapy damages endothelial cells throughout the body. However, metabolism or activation of drugs by hepatocytes results in a high local concentration. Histologically, the central venule is occluded by concentric fibrosis best shown by a trichrome Masson stain. Lesions are composed initially of von Willebrand factor, soon replaced by collagen (93). Obliteration of the central venule results in intrahepatic hypertension, diminished or reversal of portal blood flow, and ascites.
SOS/VOD, with a reported incidence of 1% to 56%, is a clinical diagnosis suggested by elevated bilirubin, weight gain, ascites, and tender hepatomegaly (94–96) (Table 76.4). The incidence variability results partly from different pretransplant conditioning regimens and the clinical criteria used to diagnose SOS/VOD. For instance, although diagnostic criteria from Johns Hopkins and Seattle seem similar, a retrospective comparison showed SOS/VOD incidence rates of 32% versus 8% (97). Risk factors for SOS/VOD include increased pretransplant liver transaminases, conditioning regimen intensity, prolonged fever, and age (95). Prior hepatitis virus exposure does not increase the risk of SOS/VOD if pretransplant liver function tests are normal. Altered drug metabolism is probably responsible for the decreased incidence of SOS/VOD in children and increased risk for SOS/VOD in persons with abnormal pretransplant liver function. Cytokines that cause fever also damage endothelial cells and probably cause the increased risk of SOS/VOD in persons with prolonged fever. In general, SOS/VOD incidence is not significantly different in recipients of allo- versus autotransplants.
TABLE 76.4 Clinical Criteria for Veno-Occlusive Disease of the Liver | |
![]() |
Clinical symptoms of SOS/VOD are also associated with many common but unrelated transplant complications. For instance, jaundice may result from hemolysis—for example, ABO incompatibility, bacterial sepsis, hepatic candidiasis, parenteral nutrition, drugs such as cyclosporine and methotrexate, or GvHD. Initial evaluation for SOS/VOD should include ultrasound of the liver, with Doppler measurement of portal vein blood flow. Reversal or diminished portal flow is consistent with intrahepatic obstruction of blood flow secondary to SOS/VOD (98); ultrasonographic findings are generally present only in overt clinical disease (99). Although uncertain cases may require liver biopsy, a percutaneous biopsy is contraindicated because of ascites, coagulopathy, and low platelets. Transjugular biopsy may, in general, be performed safely and provides an opportunity to measure the hepatic venous pressure gradient, which, if greater than 10 mmHg, is consistent with SOS/VOD (100).
Therapy for SOS/VOD is predominantly supportive. Emphasis should be on avoiding hepatotoxic drugs that will further damage the liver. Persons with severe SOS/VOD may develop hepatorenal syndrome, marked by renal insufficiency and a low fractional sodium excretion. Therapy includes diuretics to maintain baseline weight and oral ursodeoxycholic acid to lower the bilirubin and prevent further liver injury from free radicals generated by bile acids (101). Some centers attempt to maintain intravascular volume and renal perfusion with RBC transfusions, aiming for a hemoglobin of 12 to 15 g/dL. Early studies of defibrotide, a single-stranded polydeoxyribonucleotide with fibrinolytic, antithrombotic, and anti-ischemic properties, in severe SOS/VOD, suggested activity with complete response rates of 36% to 55% (102–104). No severe hemorrhage or other serious toxicity related to defibrotide was reported.
The prognosis of SOS/VOD is poor when bilirubin is more than 15 to 20 mg/dL. Thrombolytic therapy with tissue plasminogen activator and heparin has been used successfully but may be complicated by severe bleeding (105,106). Once the thrombus is replaced by fibrin and collagen, thrombolytic therapy is probably ineffective. However, in late SOS/VOD, another option is a transjugular, intrahepatic portosystemic shunt to decompress the portal vein (107–109). If there is engraftment without severe GvHD or recurrence of the underlying disorder prompting the transplant, consideration should also be given to a liver transplant (110–112).
Respiratory Failure
Transplant recipients who develop respiratory failure and require mechanical ventilation have a poor prognosis (113,114). Once endotracheally intubated, 80% of recipients are never able to be liberated from mechanical ventilation and, at 6 months, only 3% of subjects who required intubation survive. Except for procedures performed as a prelude to surgery, the reason for intubation is not correlated with a likelihood of survival, but age younger than 40 years and intubation being performed more than 100 days posttransplant correlated with better survival.
Respiratory failure within the first 30 days is usually caused by pretransplant conditioning, regimen-related epithelial cell damage, and/or infection (115–118). Early posttransplant radiotherapy and chemotherapy releases free radicals and cytokines, resulting in damage to pulmonary epithelial cells. This leads to blebs in the cell membranes, separation of junctions between cells, and necrosis, the end result being pulmonary edema, occasionally with focal or diffuse pulmonary alveolar hemorrhage (119). This may occur without an increase in pulmonary artery occlusion pressure (PAOP). Median time to the onset of alveolar hemorrhage posttransplant is about 4 months, but it may occur as late as 1 to 2 years. Symptoms are nonspecific and include dyspnea, hypoxia, and diffuse infiltrates; although hemoptysis is rare, bronchoalveolar lavage often shows intrapulmonary hemorrhage. Early in the course of respiratory distress, efforts should be directed to preventing intubation. Although not evaluated in prospective studies, and therefore of unproven benefit, management may include early invasive hemodynamic monitoring, RBC transfusions to maintain hemoglobin more than 12 g/dL, ultrafiltration to decrease intravascular volume, and anticytokine monoclonal antibodies or cytokine receptor antagonists. Use of high-dose corticosteroids is controversial but, in theory, inhibits generation of free radicals, decreases cytokine release, and decreases inflammation.
The repair process after high-dose chemotherapy and radiation may further interfere with gas exchange by causing interstitial fibrosis. Further, infection aggravates parenchymal inflammation and protracts the repair of the interstitium. Transplant recipients are especially susceptible to pulmonary infections because of bone marrow failure, immune suppressive drugs, mucositis, aspiration, and bronchial epithelial cell damage with impaired ciliary motility. Gram-negative and gram-positive pneumonias are common in the first 30 days posttransplant. Fungal infections of the lung also occur early posttransplant, and isolation of Aspergillus spp in a nasal or sputum culture should prompt initial therapy with amphotericin, voriconazole, or caspofungin. Risk factors for aspergillosis are long-term duration of impaired immunity pretransplant, for example, aplastic anemia or SCID; centers without HEPA filters to prevent inhalation of aerosolized spores; and prior invasive aspergillosis. Viral pneumonia is rare early posttransplant; the most common etiologic agent when this does occur is herpes simplex.
Heart Failure
Heart failure may result from volume overload or impairment of left ventricular function from sepsis or toxicity from pretransplant conditioning regimen drugs such as cyclophosphamide, ifosfamide, and/or anthracyclines (120–124). Pretransplant risk factors for cardiac failure are a prior history of heart failure or a low resting LVEF of less than 40% (4). Prior mediastinal radiation or a high cumulative anthracycline dose is not independent risk factors, provided the ejection fraction is normal. High-dose cyclophosphamide causes hemorrhagic myocarditis; transient ST-segment depression and T-wave inversions are common during cyclophosphamide infusion but are not a reason to alter therapy. Cyclophosphamide damages cardiac capillary endothelial cells, leading to hemorrhage between, and separation of, myocytes. The end result is loss of voltage, heart failure, and/or pericardial effusion. Unlike anthracycline-related heart damage arising from myocyte damage, even severe cyclophosphamide-induced left ventricular dysfunction is reversible after an interval of weeks to months.
Renal Failure
Renal insufficiency is usually multifactorial. Cause includes the underlying disease—for example, cast nephropathy in myeloma, prerenal decrease in glomerular filtration, intrinsic renal dysfunction or postrenal obstruction. The most common reason for renal insufficiency early in the posttransplant period is drug related, especially with use of aminoglycosides, cyclosporine, and amphotericin (125,126). Mortality in persons requiring dialysis is about 85% (127). Prerenal causes of azotemia include hepatic SOS/VOD, diarrhea, diuretics, third-spacing from sepsis, hypoalbuminemia, and a capillary leak syndrome from high-dose drugs and radiation. Hepatic SOS/VOD, like other causes of prerenal azotemia, is marked by decreased fractional excretion of sodium (FeNa+) in the urine.
Azotemia from intrinsic renal failure may result from acute tubular necrosis (ATN), glomerulonephritis, interstitial nephritis, or renal vascular damage. Causes of ATN in transplant recipients include sepsis, hypovolemia, and drugs such as aminoglycosides, amphotericin, platinums, foscarnet, and cyclosporine. In ATN, the FeNa+ is high, and the urine has muddy hyaline casts. Renal insufficiency from glomerulonephritis usually results from streptococcal or staphylococcal bacteremia. In glomerulonephritis, FeNa+ is low, and the urine sediment contains RBC casts and increased protein. Interstitial nephritis arising in the early stem cell transplantation (SCT) period is usually drug induced. Causes of allergic interstitial nephritis are penicillins, cephalosporins, sulfamethoxazole-trimethoprim, and fluoroquinolones. In allergic interstitial nephritis, the urine FeNa+ is high, and urine sediment contains WBCs, WBC casts, and eosinophils. Renal insufficiency from renovascular damage is usually caused by drugs such as cyclosporine or from hemolytic uremic syndrome (HUS), which is marked by schistocytes, thrombocytopenia, and azotemia. HUS arises from endothelial cell damage, which may be related to cyclosporine, GvHD, or high-dose drugs and radiation.
Postrenal kidney failure in transplant recipients may result from hemorrhagic cystitis with ureteral or urethral obstruction due to blood clots, retroperitoneal hemorrhage, urate nephropathy, or drugs that undergo intratubular crystallization and obstruction such as aciclovir ciprofloxacin, and triamterene. Regardless of the cause, posttransplant renal insufficiency may require a dose reduction of prophylactic immune suppressive drugs such as cyclosporine or methotrexate; this may increase GvHD.
Engraftment
Definition of graft failure is controversial. After a bone marrow graft, there is usually a rise in the WBC count by 3 weeks. After a blood cell transplant the WBC count usually rises by about 2 weeks. Platelet recovery, defined as more than 20 × 109 cells/L without transfusions typically occurs 2 to 3 weeks later. Occasionally, recipients require platelet transfusions for months posttransplant. Generally, graft failure is defined as a neutrophil count less than or equal to 0.5 × 109 cells/L by day 28. Causes of graft failure include too few normal hematopoietic cells, damage to the bone marrow microenvironment, immune-mediated graft rejection, or drug- or virus-related immune suppression (128,129).
The minimal number of bone marrow or blood cells needed for sustained engraftment is unknown. There are several reasons for this:
- It is not known which hematopoietic cell(s) are responsible for sustained engraftment.
- Different hematopoietic cells may operate under different circumstances and in different persons.
- After autotransplants there is no need for sustained engraftment because of autologous bone marrow recovery.
- There is no routinely used technique to identify the hematopoietic cell(s) responsible for sustained engraftment (130,131).
Because of these limitations, surrogate markers are used to assess the hematopoietic-restoring functionality of grafts. For instance, CD34 is a surface membrane marker of immature hematopoietic cells. In animals and humans, retrovirus-transduced CD34+ cells sometimes contribute to long-term engraftment but are not a necessary condition (132). To ensure sustained engraftment in humans, most data suggest a threshold of 2 to 4 × 108 mononuclear cells or 2 × 106 CD34+ cells/kg of recipient body weight. Autotransplant recipients receiving extensive prior chemotherapy and/or radiation frequently have fewer CD34+ cells. It may be difficult to obtain large numbers of CD34+ cells from these persons, and recipients generally recover bone marrow function later than after grafts from normal or less extensively treated donors. This may reflect decreased numbers and/or function of CD34+ cells and/or damage to the bone marrow microenvironment.
Immune-mediated graft failure is theoretically impossible after an autotransplant but it is the most common cause of graft failure for allotransplants. Risk of immune-mediated graft failure correlates with the degree of HLA-disparity between the donor and the recipient. Graft failure occurs in less than 1% after HLA-identical sibling allotransplants, 6% to 8% after unrelated HLA-matched allotransplants and in up to 20% after HLA-haplotype–matched allotransplants (133–135) (Tables 76.5 and 76.6). Immunity to non-HLA antigens, such as H-Y and KIR, also operates to increase risk of immune-mediated graft failure. Other variables influencing the risk of immune-mediated graft failure are transfusion-induced sensitization to HLA and non-HLA antigens, intensity of the pretransplant conditioning regimen and quantity of T cells in the graft. In persons with aplastic anemia, the volume of pretransplant RBC or platelet transfusions correlates with a higher rate of graft failure. This is presumed to result from sensitization of the recipient to disparate HLA and non-HLA antigens. These observations were made before microfilters were available to deplete WBC from transfused blood products. Whether this risk still operates is unknown. However, because of these considerations, potential allotransplant recipients should avoid unnecessary transfusions or receive microfiltered blood products. Removal of donor T cells from the bone marrow graft to prevent GvHD also increases the risk of graft failure; compensation may be possible by more intensive pretransplant immune suppression (136,137). Graft-failure risk is also increased after male grafts to parous and/or transfused female recipients. Here, the recipient is presumed to be sensitized to donor H-Y antigens (138,139).
TABLE 76.5 Graft-Versus-Host Disease, Graft Failure, and Disease-Free Survival for Transplants with Sibling-Matched or Unrelated Donors |
![]() |
Several bone marrow suppressive drugs commonly used posttransplant may delay and/or reverse engraftment, for example, methotrexate and sulfamethoxazole-trimethoprim. Viruses, especially herpes simplex, parvovirus, HHV-6, parvovirus-B19, and CMV, cause bone marrow suppression, possibly because they infect bone marrow stroma cells. A decline in the WBC and/or platelets after recovery posttransplant should prompt a search for a drug- or virus-related cause. Declines are also temporarily associated with tapering immune suppression; whether these are related phenomena is unclear. The effect, if any, of acute GvHD on bone marrow function is poorly understood. However, there is a clear association of decreased bone marrow function and chronic GvHD (see below). The response of chronic GvHD to immune suppression is often correlated with improved bone marrow function.
Treatment for graft failure includes using molecularly cloned hematopoietic growth factors (G- or GM-CSF), a second graft, and/or increased immune suppression. Subjects with primary graft failure (i.e., no engraftment) have a poor prognosis, whereas those with secondary or late graft failure (unsustained engraftment) do better. When graft failure is associated with reemergence of host T cells, repeat pretransplant conditioning is usually given before the second graft based on the assumption that graft failure is immune mediated; this may be incorrect in some instances. Consequently, early or late bone marrow failure should be referred to as such and not as graft rejection.
Acute Graft-Versus-Host Disease
The principal manifestations of acute GvHD are rash, diarrhea, and jaundice, present individually or in combination (140,141). Histologically, there is involvement of the basal cell layer of the skin, biliary ductules of the liver, and crypts of the distal gastrointestinal tract. Symptoms occur close to the time of engraftment but may occur earlier or at any time within the first 100 days posttransplant. Acute GvHD is an allogeneic response mediated by donor T cells, which recognizes recipient tissues as foreign. The incidence and severity of acute GvHD increase with increasing recipient age and HLA and non-HLA disparity between the recipient and the donor (142,143) (see Tables 76.5 and 76.6).
The major HLA genes are inherited from both paternal and maternal chromosome 6. The classic HLA class-1 genes are A, B, and C, and more HLA molecules are being characterized. The classical HLA class-1 molecules are present on the surface of all cells and function to present small intracellular peptides to T cells. HLA class-2 molecules are DR, DP, and DQ. These surface molecules present extracellular peptides that result from endocytosis of extracellular protein and degradation of these proteins into smaller peptides (144,145). Even after an HLA genotypically matched allotransplant, acute GvHD invariably develops when—usually inadvertently—no posttransplant immune suppression is given. This likely arises because of recognition of host-derived peptides presented by HLA molecules and recognized as foreign by donor T cells (146).
Skin involvement in acute GvHD results in a maculopapular, erythematous rash, often beginning on the palms and soles and which may become systemic. In severe cases, acute GvHD with skin involvement may be pruritic with bullae. The skin involvement in acute GvHD may be precipitated by exposure to sunlight and/or drugs. Histologically, one can see the dermal–epidermal border disrupted by vacuolar degeneration of epithelial cells, dyskaryotic bodies, acantholysis (i.e., separation of cell–cell contact), epidermolysis (separation of the epidermal and dermal layers), and lymphocytic infiltration. These clinical and histologic findings are not unique to acute GvHD and may occur from drug allergy or the effects of the high-dose chemotherapy and radiation used in the pretransplant conditioning regimen.
Gastrointestinal involvement with acute GvHD results in diarrhea, often accompanied by cramping abdominal pain. In severe cases, the diarrhea may be bloody or associated with a paralytic ileus. Histologically, lymphocytes and apoptotic cells are present and intestinal crypts are lost, which leads to epithelial denudation. Evaluation of gastrointestinal tract signs and symptoms should include stool cultures for bacteria, fungi, and viruses, especially CMV. Sigmoidoscopy with biopsy may be helpful if the diagnosis is in doubt and platelet levels are sufficient. Acute GvHD with hepatic involvement presents as jaundice and an elevated alkaline phosphatase with or without elevated transaminases. The differential diagnosis includes SOS/VOD or infections with CMV or Candida spp and may require a transjugular liver biopsy for accurate diagnosis. In acute GvHD, the liver biopsy may show T-cell infiltration of the portal triad, with apoptosis of epithelial cells lining the biliary tree.
TABLE 76.6 Incidence of Grade III–IV Acute Graft-Versus-Host Disease in CML According to the Number of Mismatched Class I and Class II Alleles |
![]() |
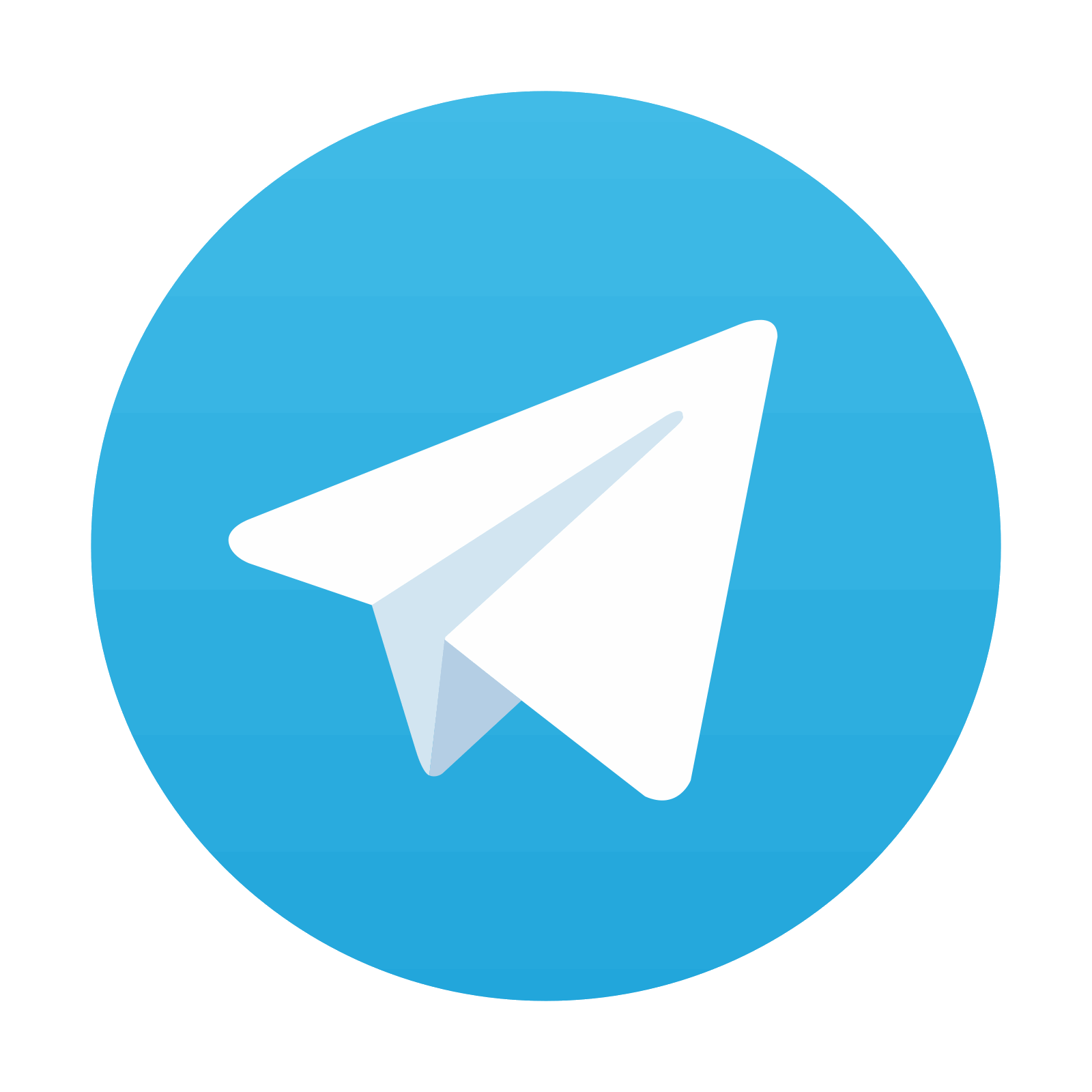
Stay updated, free articles. Join our Telegram channel
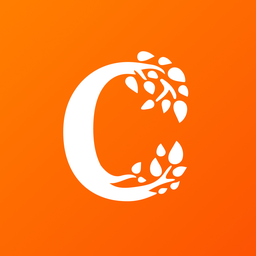
Full access? Get Clinical Tree
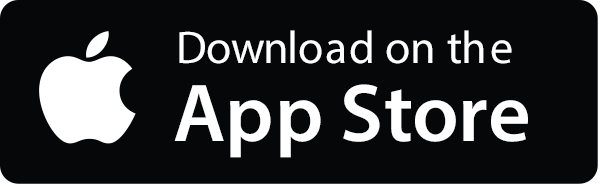
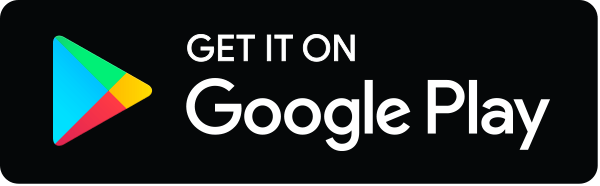