The hepatic lobule is the anatomical and functional unit of the liver. It has the shape of a polygonal (hexagonal) pyramid with an apex trunk of 1 mm in diameter and a height of about 1.5–2 mm, delimited by a layer of connective tissue (reticular connective). Each lobule is formed by numerous cellular laminae consisting of hepatocytes; the plates are perforated and anastomosed with each other and define a system of irregular vascular spaces: the hepatic sinusoids. The cellular edges and capillaries show a radial arrangement converging from the periphery toward the centre of the lobule. The axis of the lobule is occupied by the centrilobular vein, tributary of the hepatic vein, within which the sinusoids open up. The area where three or more adjacent lobules are in contact with each other is called a portal space (‘portal triad’, containing a bile duct, a terminal branch of the hepatic artery and the portal vein). Metabolic zones form the hepatic acinus [2]. Zone 1 is the periportal zone, is centred around the portal triad and, being close to the hepatic artery, is oxygen rich. It is more resilient to hemodynamic stressors, least susceptible to necrosis and first to regenerate. These zones are involved in gluconeogenesis/glycogenolysis. Zone 3 is the pericentral or perivenous zone, very close to the central vein: it has the lowest oxygen tension. This zone is highly susceptible to stressors, hypoxia and is the last to regenerate. Zone 3 is the site of drug detoxification and phase 1 and 2 metabolism [2].
The liver has a dual blood supply, which includes an arterial system (hepatic artery, HA) and a venous system (portal vein, PV). The total liver blood flow is approximately 1,500 ml/min (100 ml/min/100 g). The hepatic artery flow is approximately 300 ml/min (30 % of total hepatic flow, 45–50 % of total oxygen intake), while portal vein is usually estimated between 1,000 and 1,200 ml/min (70 % of total blood flow, 50–55 % of oxygen supply). The O2 hepatic extraction, normally less than 40 % (4–6 ml/100 g/min), is increased in case of increased demand [2].
Both the terminal portal venules and the terminal hepatic arterioles end in the hepatic sinusoids, through which the entire afferent hepatic blood flows before reaching the efferent vascularisation (terminal hepatic venules). Hepatic blood drainage is via the hepatic veins (right, left, middle) which drain into the inferior vena cava.
The portal system is a low-pressure (5–12 mmHg)/low-resistance system: the gradient between portal vein and hepatic vein (HVPG) ranges between 1 and 5 mmHg: portal hypertension is defined as a pressure of >12 mmHg or for a portal vein–inferior vena cava (IVC) gradient >5 mmHg. The arterial system has a high level of resistance and a high flow. Hepatic blood flow and distribution are dependent on hepatic arterial resistance, intrahepatic portal resistance and portal flow. Intrinsic and extrinsic mechanisms regulate the hepatic blood flow. Among the extrinsic factors, sympathetic innervation plays an important role in controlling vascular tone (adjusting the capacitance of the uptake capacity of circulating blood). Within the hepatic arterial bed, both alpha (alpha 1 and 2) and beta (beta 2) receptors are represented, while only alpha receptors are present in the portal vein. Dopaminergic receptors are present in both vessels. Vasopressin, able to increase the hepatic artery resistances, reduces the PV resistances, thus playing a relevant role in the treatment of portal hypertension. Maintaining a constant hepatic inflow is crucial to optimise drug metabolism and synthetic functions. Self-regulating mechanisms, present in the hepatic artery, are not demonstrated in the portal vein. Among the intrinsic factors (working independently of humoral neuroregulation) are self-regulating mechanisms for flow/pressure: in case of critical drop in blood pressure, arterial hepatic blood flow is maintained until systolic arterial pressure falls below 75–80 mmHg, via reduction of resistance mechanism. In case of reduced portal flow, hepatic artery flow increases, buffering the changes in portal flow (hepatic artery buffer response, HABR). In a situation of critical drop in portal flow (flow reduction of 20–30 %), there is an increased vicarious flow in the hepatic artery (increase up to 100 %) in order to maintain the hepatic blood supply. The mechanism is supported by the reduction of adenosine washout (present with normal portal flow, but missed in the presence of low flow) followed by hepatic artery vasodilatation. A portal flow counterbalance in the presence of hepatic artery occlusion does not seem to exist. Instead, there is an increased hepatic arterial resistance with flow reduction in case of elevated hepatic venous pressure [1, 2].
The liver performs many functions such as protein synthesis; uptake, storage and metabolism (biotransformation and degradation) of endogenous and exogenous substances (among them carbohydrates, lipids, proteins, hormones, drugs); bile production; and immune defence with different mechanisms, mainly operated by the Kupffer cells (KC, so-called fixed hepatic macrophages, 80–90 % of the reticular endothelial system, 10 % of the entire liver cell mass), responsible of phagocytosis (bacteria, fungi, viruses, immune complexes, antigens), clearance of endotoxin and secretion of mediators capable of adjusting microcirculatory hepatic protein catabolism. Due to the indeed wide variety of processes the liver is involved, no single parameter or test able to measure all components simultaneously has been available so far [1–4].
13.2 Static and Dynamic Liver Function Tests [1–5]
To assess liver function, static (also called conventional tests) and dynamic tests are available [3–5]. The static tests track the different functions separately, and, in the event of injury, they might describe its size: these tests are, since long, part of scoring systems, able to track chronic (Child–Pugh; MELD) or acute (SOFA) functional impairment of the liver. However, static tests are not able to predict the quality and the extent of the functional recovery of the liver (or of a newly grafted liver), as they essentially show a ‘frozen’ (static) representation of the integrity (or not) of hepatocytes and of metabolic and synthetic pathways (Fig. 13.3). Metabolic function includes enzymes of the cytochromes (phase 1) and the phase of glucuronide conjugation (phase 2). Bilirubin, derived from haemoglobin catabolism, expresses the capacity of uptake, conjugation and excretion in the bile of haeme (breakdown product of haemoglobin; 1 g Hb leading to 36 mg bilirubin). After uptake by the liver cell, the haeme is exposed to glucuroconjugation (metabolic phase 2) for solubilisation, aimed at excretion in the bile: the process can be relatively insensitive to ischaemic insult, at least in the early stages. In general, hyperbilirubinaemia depends on haemolysis (pre-hepatic disease), cell damage or reduced intrahepatic bile excretion (cholestasis). Albumin, vitamin K-dependent coagulation proteins (factors II, VII, IX, X; protein C; protein S; and protein Z), factor V, fibrinogen, antithrombin, alpha 2 plasmin inhibitor, and plasminogen represent the (elevated) share of synthetic activity of the liver. The short half-life of FV (4 h) and FVII (4–6 h) might quantify the liver damage in acute liver failure and is used in the Clichy criteria, together with the presence of hepatic encephalopathy, to indicate LTx for FHF [6].


Fig. 13.3
ICG indicator dilution curve. ICG indocyanine green, C ICG, ICG blood concentration. A Primary peak, B secondary peak (recirculation phase), C (hepatic) elimination phase (From Vos et al. [9])
13.3 Liver Enzymes, Liver Function and Liver Injury [4, 5]
AST (aspartate aminotransferase) and ALT (alanine aminotransferase), present in various organs, have an important role for the metabolism of amino acids. AST is less specific, being present at cardiac and muscular level. ALT is more liver specific, is highly represented in the cytoplasm and is, for the most part, in the periphery of the liver lobule: moderate increase is reported in case of centrilobular hypoxia. In the presence of acute hepatic injury (acute hepatitis), the serum concentration of ALT, mainly represented in the periportal areas of the liver, increases significantly, as a consequence of increased permeability of the cell membrane or necrosis. Increased AST/ALT is associated with ischaemic injury and its size (liver injury), but does not provide information on functional hepatic impairment and therefore is considered inferior to dynamic tests when assessing hepatic functional reserve [3–5]. Alkaline phosphatase (ALK) and gamma-glutamyl transferase (GGT) are mainly used to quantify cholestasis. Lactate dehydrogenase (LDH, mainly fraction 5) is a rather nonspecific index of ischaemic liver (but not only!).
Dynamic tests [3, 7] are related to the ability of the liver to metabolise or eliminate substances. Unlike conventional tests, dynamic tests are able to assess liver function within a relatively short time span, are repeatable in relatively short time and may confidently provide a reliable and more global prognostic assessment (Fig. 13.2). Among them are ICG clearance, caffeine test, BSF clearance, amino acid clearance, galactose elimination capacity, formation of monoethylglycinexylidide from lignocaine (MEGX test) and aminopyridine test.
The aminopyrine breath test measures the microsomal metabolical capacity of the liver cell. The test is based on demethylation and subsequent metabolism of 14C-aminopyrine marked by the microsomal enzyme cytochrome P450 dependent. The extent of demethylation is measured indirectly by the exhaled CO2.
Galactose elimination capacity (depending on cytosolic mitochondrial function) studies the function of the liver cell. Metabolisation occurs through phosphorylation by the galactokinase. The test is complex in clinical practice since it requires samples over 20–50 min and presents false positives in relation to fasting and liver regeneration.
Monoethylglycinexylidide (MEGX) is a derivative of lidocaine metabolism and is related to the activity of cytochrome P450. It has been used in the past (and still by some) in liver surgery (value >25 ng/ml predicts safe liver resection) and as a prognostic indicator in patients candidate for liver transplantation. The results can be altered by interaction with other drugs that affect the cytochrome P450. The test, performed in the laboratory and whose results are not obtained in real time, has not been used in the field of anaesthesia and ICU.
13.4 The Clearance Principle: Liver Function and Hepatic Perfusion [8]
Most quantitative liver function tests rely on the clearance principle: the hepatic clearance (Cl) is the product of flow (Q) and hepatic extraction (Ex).


(13.1)
According to this principle [8], substances are classified at high or low hepatic extraction rate. In case of drugs at high extraction rate, liver blood flow becomes the limiting factor, and clearance will approach liver blood flow. Instead, low extractable compounds are flow independent: in this case, the hepatic clearance is the measure of the metabolism and/or elimination process, or more precisely, it represents the intrinsic ability of the liver to remove substances without flow limitation, i.e. the intrinsic hepatic clearance. Equation 13.1 can be rewritten as


(13.2)
Then, according to Imamura et al. [8], the measure of clearance of compounds with high extraction rate is an indicator of liver blood flow, while the clearance of low extractable substances represents intrinsic clearance (Clint). In healthy subjects, with very high extraction, indocyanine green (ICG) clearance is considered a surrogate of hepatic blood flow; on the contrary, in cirrhotic patients, due to the reduced hepatic extraction secondary to the disease, ICG clearance represents the intrinsic clearance, characterised by a reduced uptake: steady-state hemodynamic conditions are mandatory to adequately assess liver function.
13.5 Indocyanine Green Clearance: The Tests
Among the dynamic tests, indocyanine green (ICG) clearance remains the most relevant, widely used in clinical practice today both in the surgical and medical critically ill patient [9–12]. ICG is a fluorescent, water-soluble, inert, tricarbocyanine with a very high hepatic extraction (70–80 %) and a very low toxicity (the side effects described are very rare, about 1/40,000): the only known contraindications are allergy (non-immunological histamine release), iodine allergy or thyrotoxicosis, the latter due to the presence of iodine in the molecule. Since 1960, its elimination rate has been largely used to measure liver function and hepatic blood flow [13–15]: in the late 1990s, Lau had shown the superiority of ICG clearance (ICGCl) compared to the aminopyrine breath test and the clearance of amino acids as a predictor of mortality in patients undergoing liver resection [15]. Important applications are now available in liver resection surgery, in liver transplantation and in intensive care [3, 9–11]. The conventional measurement of ICGCl requires serial blood sampling after ICG injection. Ex vivo photometric analysis of consecutive arterial blood samples after intravenous bolus injection is the gold standard; however, being a time-consuming and complex procedure, it has now been abandoned in favour of the more recently developed monitors that allow transcutaneous noninvasive pulse dye densitometry (PDD) tests at the bedside (LiMON, Pulsion, Germany; DDG-2001 Nihon Kohden, Japan) [9–11]. Available monitors express ICG elimination in terms of the ICG plasma disappearance rate (ICGPDR) or retention rate at 15 min (ICGR15), since only relative ICG concentration changes have to be assessed (Fig. 13.3).
The results of ICG elimination measured by the PDD method have been shown to correlate with those obtained by the invasive method used in critically ill patients (whether or not haemodynamically stable) and in patients after liver surgery. Both devices calculate the rate constant (k) of the ICG indicator–dilution curve using backward dynamic extrapolation of the elimination phase [9]. Appropriate calculations using K value allow the determinations of functional parameters able to quantify liver function. The ICG kinetics have been studied since 1960 in animals and humans to measure blood flow, cardiac output and circulating blood volume and, later, to assess liver function [9–11]. Due to high hepatic extraction (70–80 %), ICG clearance has been used as an index (measure) of liver blood flow. The usual ICG dose of 0.5 mg/kg generates in the average subject an initial plasma concentration of 100 mg/ml: according to Sakka, however, reliable results are also available using 0.25 mg/kg [3]. Once injected, ICG shows a very high protein binding (95 % to albumin, alpha1 lipoproteins and beta lipoproteins) and a very short half-life (3–5 min) [9–11]. It distributes uniformly and rapidly (2–3 min) in the blood, with a volume of distribution approximately equal to that of plasma. ICG is almost entirely extracted by the liver (uptake across the sinusoidal plasma membrane) and transported within the liver cells by transporting polypeptides (1 B3 and Na-taurocholate cotransporting polypeptides). It is excreted unchanged almost exclusively into the bile in nonconjugated form with neither metabolism nor enterohepatic recirculation, carried by the ATP-dependent export pump multidrug resistance-associated protein 2 (MDRP2) and multidrug resistance P-glycoprotein (MDR3), reflecting hepatic excretory function and hepatic energy status [9–11]. Then two processes are involved in hepatic ICG clearance: sinusoidal uptake and canalicular excretion, the former playing the most important role in humans. Due to the very high hepatic extraction, ICG clearance is, in normal conditions, hepatic blood flow limited. After the intravenous bolus administration, the ICG dilution curve shows (i) a primary peak (used to calculate cardiac output); (ii) a second elimination peak (recirculation phase), sometimes followed by smaller peaks, used to estimate circulating blood volume; and (iii) an (hepatic) elimination phase, lasting 10–20 min [9].
In the dye dilution time curve, two components are recognised [8]: (a) the distribution phase, representing ICG removal from the plasma, due to the uptake by the liver cells; and (b) the elimination phase in the bile (97 % of the entire elimination process) (Fig. 13.4).
The decay is bi-exponential, with linear kinetics up to a dose of 1 mg/kg. The ICG hepatic clearance therefore depends on hepatic blood flow and transporter capacity: signs of liver dysfunction as indicated by impaired clearance of ICG (see below) may depend on reduction of blood flow, on impaired cellular function, or on both (blood flow clearance and transporter capacity). ICG half-life is substantially prolonged in the case of liver disease. Different from the usual two-compartment model, ICG is excreted from the peripheral compartment (the liver) and not from the central compartment (plasma). According to this model, the initial rapid fall in concentration, called the distribution phase, represents the uptake of ICG from the plasma by the liver; the subsequent relatively slow fall, called the elimination phase, represents the elimination of ICG from the liver into the bile. The transition from the distribution phase to the elimination phase of ICG occurs approximately 20–30 min after the administration. ICGK (min−1) is usually determined from the first 15-min component of the ICG disappearance curve.
The ICG transport capacity may be reduced either because of the downregulation of organic anion-transporting polypeptides [4] or by competitive inhibition due to hyperbilirubinaemia [16]. With respect to the former, cytokines such as tumour necrosis factor-α (TNF-α) and interleukin 6 (IL6), released by Kupffer cells in patients with steatosis and/or hepatic injury (hepatitis), are able to affect the expression of organic anion-transporting polypeptide isoforms and sodium taurocholate cotransporting polypeptide, thereby affecting ICG uptake by the liver. Bilirubin and ICG share the same enzyme transport system (ATP export pump MDRP2), making high the chance of altered results (false pathological values) in the presence of hyperbilirubinaemia (vide infra) [9, 16, 17]. Consequently, the ICG test may be of limited value in patients with a condition of global hepatocellular dysfunction and in the case of hyperbilirubinaemia (generation of false poor results) [9, 10]. At the opposite site are high flow states, able to mask an altered excretory function and providing false-reassuring results (better than expected liver function), due to the generation of false ‘normal or near-normal’ results [10]. It has been documented that ICGCl is not adequate to measure hepatic blood flow in specific clinical settings: for example, the fraction of hepatic extraction (70–80 % in healthy subjects) is greatly reduced in cirrhotic patients (20–30 %), making ICGCl a representation of Cint (uptake clearance; see above) [18]. Studying ICG kinetics in patients with cirrhosis, Kawasaki et al. [19] reported significant alterations of uptake and release constants (k) (but the release constant is questioned by other authors). Instead, the constant of elimination of ICG in the bile seems to be normal. In the same study, measuring hepatic blood flow with the galactose clearance test, it was shown that in cirrhotic patients, the reduction of ICGCl, expressed as ICGR15 (the circulatory retention of ICG 15 min after bolus injection, vide infra), does not (only) depend on hepatic blood flow reduction but also on a reduced extraction of the liver cells. In cirrhotic patients, this phenomenon seems to be related to the extent of the sinusoidal capillarisation and to the presence of intrahepatic shunts [9, 19, 20]. In normal conditions, substances (including proteins) diffuse freely between the sinusoids and hepatocytes. As sinusoid capillarisation develops, diffusion of these substances becomes impaired and barrier limited, as is in the capillaries of other districts. Due to its high protein binding, ICG is very sensitive to the sinusoidal capillarisation. Figure 13.6 shows the disappearance of ICG from plasma in normal subjects (full signs) and cirrhotic subjects (empty signs). According to Imamura, in cirrhotic patients, ICGK and ICGR15 might reflect the degree of sinusoidal capillarisation, intrahepatic shunts and, in part, reduced liver blood flow [19].
The logarithmic transformation of the curve in the distribution phase allows the measurement of different parameters that quantitatively assess the removal of ICG by the liver cells [9, 10].
1.
Constant K (ICGK) – disappearance rate constant or elimination rate constant
2.
Clearance of green indocyanine (ICGCl)
3.
Plasma disappearance rate (ICGPDR)
4.
Retention rate at 15 min (ICGR15)
In clinical practice, ICGPDR and ICGR15 are the ICG kinetic parameters most frequently used to dynamically assess liver function [9, 10] (Fig. 13.5):
1.
ICG PDR – plasma disappearance rate
(a)
Reduction of ICG blood concentration expressed as the percentage change over time, starting (time 0) from a concentration of 100 % (normal values >18 %/min). The method, having satisfactory correlation (r 2 = 0.77) with ICGCl, is validated as a surrogate for clearance in the critically ill)
(i)
PDR(%/min) = ln 2/t½ × 100 ICGPDR might represent, at variance of ICGR15, ICG uptake by hepatocytes, its excretion into the bile, blood flow-dependent liver metabolism and energy status [21]
2.
ICGR15 – retention ratio after 15 min
(a)
The relationship between the concentration of ICG at 15 min and initial concentration (normal <10 %)
(i)
R15 (%) = C ICG15/C ICG T0 × 100
Assuming an initial plasma concentration of 100 mg/ml (0.5 mg/kg ICG in a subject with a plasma volume of 50 ml/kg BW), ICGR15 can be determined by transforming the ICG concentration curve to a ‘point zero’ (100 %) and then describing the decay (at min 15) as percentage change per time (%/min) in a logarithmic graph, seen as a negative slope. According to Imamura, as the assumption of the initial concentration of ICG is correct, ICGR15 is pharmacologically equivalent to ICG K, and it has been widely used as an alternative to ICGK for its convenience [8]. It might represent the hepatic blood flow.
3.
ICG plasma clearance 500–700 ml/min/m2
(i)
It depends on hepatic blood flow, liver function and bile flow. It describes the volume of blood completely purified by ICG in the time unit.
ICGPDR and ICGR15 are the two sides of the same phenomenon: ICGPDR reads the disappearance of ICG from plasma (% per min) and ICGR15 the amount of ICG remaining in circulation 15 min after the administration. However, these tests are used in the literature in a different (and potentially confounding) manner. ICGR15 is mainly used in the evaluation of the functional reserve of the liver in case of hepatic resection in cirrhotic patients [8, 11], ICGPDR and ICG R15 in the assessment of liver graft function [22] and PDR and clearance in critically ill patients [3, 21].
As mentioned above, plasma disappearance rate (ICGPDR) and retention rate at 15 min (ICGR15) are calculated using either the conventional invasive method or, more recently and more simply, a noninvasive method (pulse dye densitometry (PDD) and spectrophotometry) [9–11]. The conventional invasive method involves the construction of the dilution curve by measuring the concentration of ICG in plasma by spectrophotometry of various arterial blood samples taken at defined times: it has been used in many studies dealing with functional assessment of the liver even in recent times [19]. However, this method is expensive and difficult to implement in clinical practice. In the 1990s, it was possible to measure PDR through a fibre-optic catheter placed in the femoral artery and connected to a computer (COLD System, Pulsion) which allowed, together with the measurement of PDR, the study of volumetric haemodynamics [23]. As already mentioned, the noninvasive method relies, instead, on the measurement of ICG concentrations using PDD and spectrophotometry via an optical transcutaneous pulse spectrophotometric sensor (DDG-2001 Nihon Kohden; LiMON, Pulsion) [11, 24–26]. Pulse dye densitometry measures the concentration of ICG in blood based on the principle of the difference in absorption of the light wave with two different frequencies: 805 nm (absorption frequency of the peak of ICG) and 905 nm (frequency at which ICG has no absorption); the principle is analogous to the mechanism of absorption of the difference between oxyhaemoglobin and reduced haemoglobin. There is no influence of haemoglobin, whether oxidised or reduced, absorption being very low at the frequency wave used for ICG. The same is true for bilirubin (absorption peak at 470 nm) [9, 10]. This method has been validated both in conditions of haemodynamic stability in critically ill patients as well as in unstable conditions, such as in liver transplantation [22–25]. Both systems have algorithms which calculate the constant K (‘rate constant’) using an equation which deals with the elimination phase (‘backward dynamic extrapolation’) [9]. The ICG kinetic parameters are determined by monoexponential transformation of the original ICG concentration curve, backward extrapolation to the time point ‘zero’ (100 %), and describing the decay as percentage change per time [9]. The algorithm was validated by Purcell et al. determining ICGR15 with LiMON and direct measurement of blood samples [26]. The most recent revisions on this item insist on the need for haemodynamic stability in order to obtain reliable data on liver function [9, 11]. In fact, as already underlined, hepatic blood flow and cellular uptake are variables able to impact on ICG elimination (increasing or decreasing the elimination). The hepatic blood flow can be altered by systemic factors (reduced cardiac output able to impact on the hepatosplanchnic perfusion) or by local factors (thrombosis of the hepatic artery or abdominal hypertension); both tend to reduce ICG elimination. On the contrary, the possibility exists that a splanchnic hyperperfusion results in a rise in the extraction of ICG and therefore in a (false) increased PDR.
True pathological ICGPDR or ICGR15 is present in the case of end-stage liver failure (cirrhosis) or of severe rejection after liver transplantation, both conditions able to reduce ICG liver extracting abilities [9, 10]. Elevated ICGR15 in cirrhotic patients may be due to:
1.
Decreased ICG transport from the systemic circulation to the liver
2.
Decreased uptake from the sinusoids into hepatocytes
Altered ICGPDR and ICGR15 are present also in case of cholestasis: as mentioned above, ICG and bilirubin, competing for the same carrier in the transport process in hepatocytes (sodium taurocholate cotransporting peptide), can alter ICG elimination parameters (by competition and not by functional defect). This condition, not uncommon in the early postoperative period of liver transplantation, can be associated with falsely pathological results, leading to misinterpretation of the tests. Generally speaking, the pathological results may be wrongly attributed to a condition of liver dys/malfunction (falsely reduced function) which might not be the real condition: falsely altered ICGR15 or ICGPDR is present when serum bilirubin level is >3 mg/dl [9, 10]. Thus, extreme caution must be used interpreting ICG tests in the presence of hyperbilirubinaemia. In the literature, bilirubin level above 3 mg/dl is reported as the cut-off value: in AA’s personal experience in a series of liver-transplanted patients, the cut-off should be at 6 mg/dl [27].
ICGPDR and ICGR15 can be used as a starting point for preoperative screening and evaluation of the functional reserve in the case of liver resection, as proposed by Makuuchi’s group [8]; with sequential assessments during liver transplantation or during the early postoperative period of liver transplant (OLT) (functional recovery of the transplanted liver); after hepatic resection (functional assessment of the remnant liver after hepatic resection, also in case of living-related donation). In both cases, mandatory is caution in interpreting the results in the presence of hyperbilirubinaemia, frequent in post-liver transplantation and able to give false/altered information on ICG results (high R15/low PDR) [27]. Finally, too short intervals between sequential ICG administrations (<30 min) might significantly alter ICG clearance parameters: this phenomenon is correlated with a baseline drift associated with residual circulating ICG, possibly leading to an (incorrectly) increased ICGPDR [9]. Clinical indications for ICG elimination in perioperative liver medicine and ICU are the following [3, 9–11]:
1.
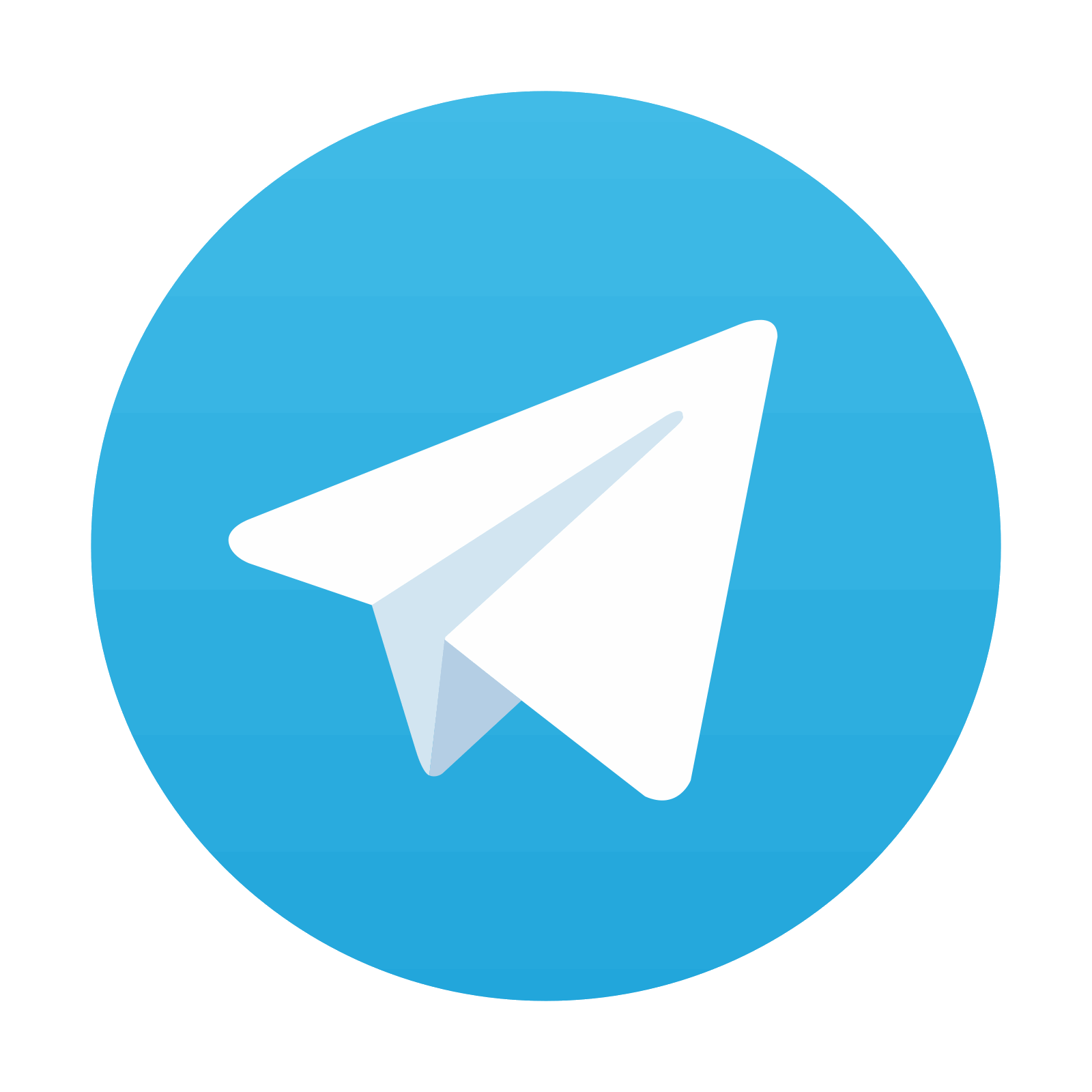
Assessment of hepatic functional reserve and prediction of mortality and morbidity in hepatic resection in cirrhotic patients
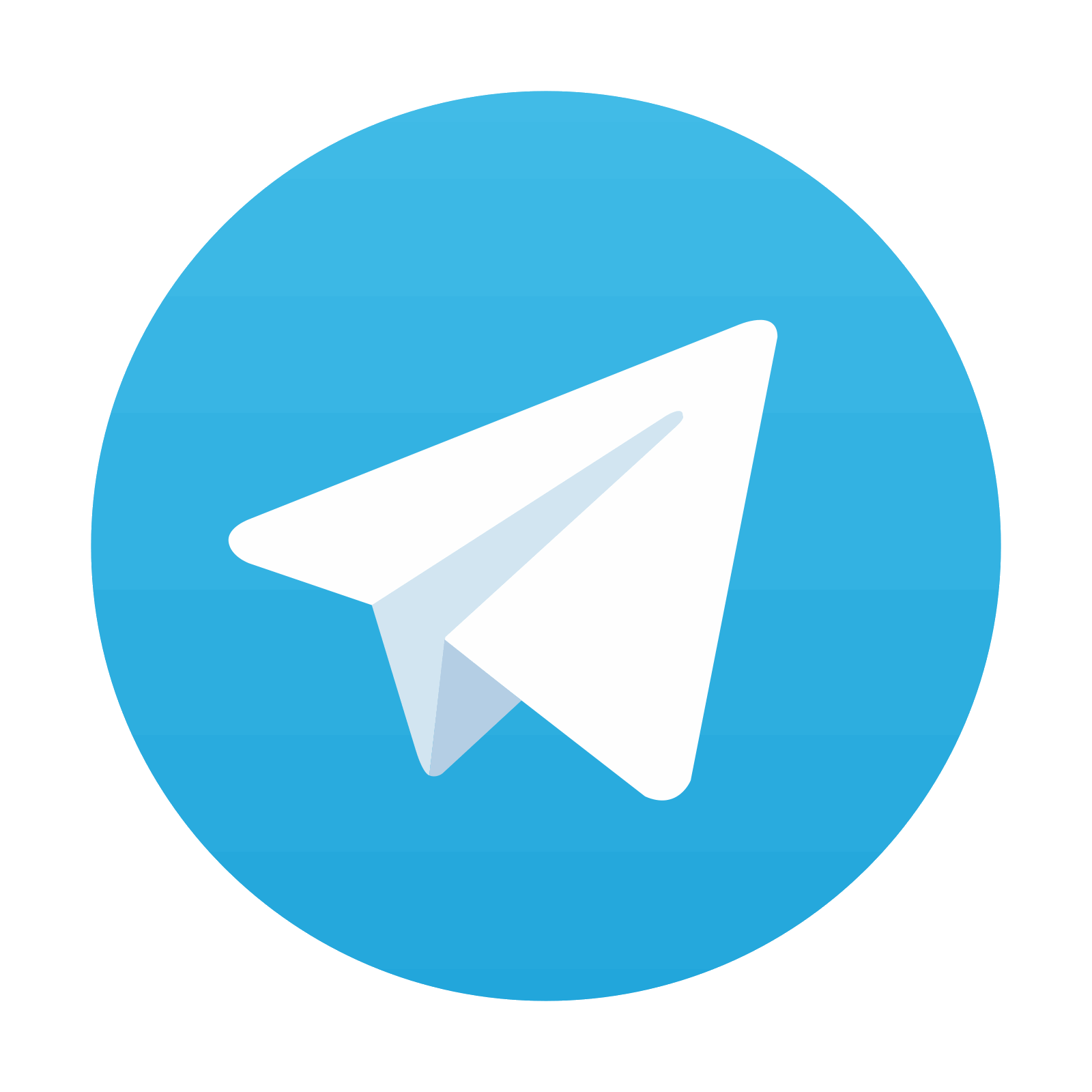
Stay updated, free articles. Join our Telegram channel
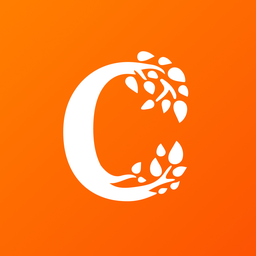
Full access? Get Clinical Tree
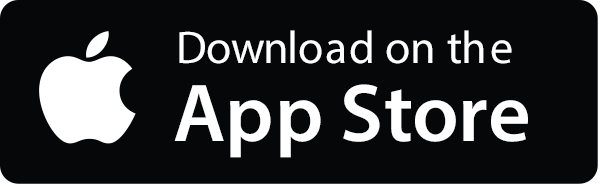
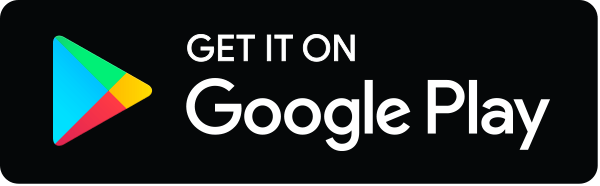
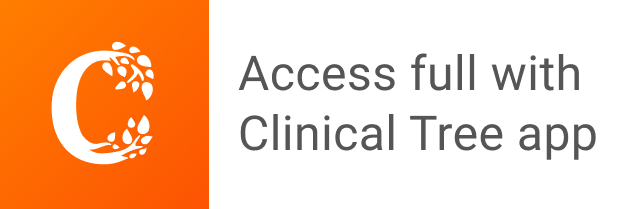