No specialty in medicine manages cardiac and pulmonary physiology as directly on a daily basis as anesthesiology. An understanding of cardiorespiratory physiology prepares the anesthesia team to manage critical and common situations in anesthesia, including hypotension, arterial hypoxemia, hypercapnia, and high peak airway pressures.
Hemodynamics
Arterial Blood Pressure
Systemic arterial blood pressure and mean arterial pressure (MAP) are commonly monitored by anesthesia providers via a blood pressure cuff or an indwelling arterial cannula. Although treatment of chronic systemic hypertension is sometimes necessary, acute hypotension is often a problem with many anesthetics. Hypotension varies from mild clinically insignificant reductions in MAP from general anesthesia or regional anesthesia to life-threatening emergencies. Hypotension can be of sufficient magnitude to jeopardize organ perfusion, causing injury and an adverse outcome. Organs of most immediate concern are the heart and brain, followed by the kidneys, liver, and lungs. All have typical injury patterns associated with prolonged “shock.” Understanding the physiology behind hypotension is critical for diagnosis and treatment.
Intraoperative hemodynamic instability has long been thought to result in worse outcomes after surgery. In recent large retrospective studies, intraoperative hypotension of even 5 minutes’ duration (systolic blood pressure [SBP] < 70 mm Hg, MAP < 50 mm Hg, diastolic blood pressure [DBP] < 30 mm Hg) is associated with increased postoperative morbidity and mortality risks. In addition, the combination of hypotension, small volatile anesthetic concentrations, and low bispectral index scale (BIS) values have been associated with worse postoperative outcomes. Whether a change in anesthetic management will alter these risks needs future study.
Physiologic Approach to Hypotension
The logical treatment of acute hypotension categorizes MAP into its physiologic components:
MAP=SVR×CO
MAP=SVR×CO
MAP=SVR×CO
Although most of our focus is on understanding MAP alone, the other pressures (e.g., SBP, DBP, and pulse pressure [PP = SBP – DBP]) also require attention. The pulse pressure is created by the addition of stroke volume (SV) on top of the DBP within the compliant vascular tree. The aorta is responsible for most of this compliance. Increased pulse pressure can occur with an increased SV but most often occurs because of the poor aortic compliance that accompanies aging (also see Chapter 35 ). Decreasing DBP can have more dramatic effects on SBP when vascular compliance is poor.
Systemic Vascular Resistance
Most drugs administered during general anesthesia and neuraxial regional anesthesia (also see Chapter 17 ) decrease SVR. Several pathologic conditions can produce profound reductions in SVR, including sepsis, anaphylaxis, spinal shock, and reperfusion of ischemic organs. The calculation for SVR follows:
SVR=80×(MAP−CVP)/CO
SVR=80×(MAP−CVP)/CO
SVR=80×(MAP−CVP)/CO
Pulmonary artery (PA) catheterization can be used to obtain the measurements necessary for calculating SVR, but this monitor is not usually immediately available. Signs of adequate perfusion (e.g., warm extremities, good pulse oximeter plethysmograph waveform, and perfusion index ∗ ) may sometimes be present when hypotension is caused by low SVR. On the other hand, hypertension nearly always involves excessive vasoconstriction.
∗ Perfusion index is a measure of the pulsatile signal relative to the background absorption and is an important measure of signal strength.
Resistance is inversely proportional to the fourth power of the radius. Individually, small vessels offer a very high resistance to flow. However, total SVR is decreased when there are many vessels arranged in parallel. Capillaries, despite being the smallest blood vessels, are not responsible for most of the SVR because there are so many in parallel. Most of the resistance to blood flow in the arterial side of the circulation is in the arterioles.
Cardiac Output
As a cause of hypotension, decreased CO may be more difficult to treat than decreased SVR. Increased CO is not usually associated with systemic hypertension, and most hyperdynamic states, such as sepsis and liver failure, are associated with decreased systemic blood pressure.
CO is defined as the amount of blood (in liters) pumped by the heart in 1 minute. Although the amount of blood pumped by the right side and left side of the heart can differ in the presence of certain congenital heart malformations, these amounts are usually the same. CO is the product of heart rate (HR) and SV, the net amount of blood ejected by the heart in one cycle:
CO=HR×SV
CO=HR×SV
CO=HR×SV
CO can be measured clinically by thermodilution via a PA catheter and by transesophageal echocardiography (TEE). Less invasive devices to measure CO have been developed, including esophageal Doppler and pulse contour analysis. Because the normal CO changes according to body size, cardiac index (CO divided by body surface area) often is used.
Heart Rate
Tachycardia and bradycardia can cause hypotension if CO is decreased. The electrocardiogram (ECG), pulse oximetry, or physical examination can identify the presence of bradycardia or tachycardia. The identification of a P wave on the ECG is essential for analyzing HR. Loss of sinus rhythm and atrial contraction results in decreased ventricular filling. Atrial contraction constitutes a significant percentage of preload, even more so in patients with a poorly compliant ventricle. A slow HR may result in enhanced ventricular filling and an increased SV, but an excessively slow HR results in an inadequate CO. Tachycardia may result in insufficient time for the left ventricle to fill and result in low CO and hypotension.
Ejection Fraction and Stroke Volume
Ejection fraction (EF) is the percentage of ventricular blood volume that is pumped by the heart in a single contraction (SV/end-diastolic volume [EDV]). Unlike SV, the EF does not differ on the basis of body size, and an EF of 60% to 70% is considered normal. Hyperdynamic states such as sepsis and cirrhosis are reflected by an increased EF. Poor cardiac function is indicated by a small EF. Because CO can be maintained by increasing HR, the SV should be calculated to better assess cardiac function. However, with chronic dilated cardiomyopathy, the SV can improve despite the smaller EF.
Preload
Preload refers to the amount the cardiac muscle is “stretched” before contraction. Preload is best defined clinically as the EDV of the heart, which can be measured directly with TEE. Filling pressures (e.g., left atrial [LA] pressure, pulmonary capillary wedge pressure [PCWP], pulmonary artery diastolic [PAD] pressure) can also assess preload. CVP measures filling pressures on the right side of the heart, which correlates with filling pressures on the left side of the heart in the absence of pulmonary disease and when cardiac function is normal. By using a balloon to stop flow in a PA, pressure equilibrates within the system so that PCWP is nearly equivalent to LA pressure and reflects the filling pressure of the left side of the heart. The relationship between pressure and volume of the heart in diastole is depicted by ventricular compliance curves ( Fig. 5.1 ). With a poorly compliant heart, normal filling pressures may not produce an adequate EDV. Likewise, trying to fill a “stiff” left ventricle to a normal volume may increase intracardiac and pulmonary capillary pressures excessively.

Frank-Starling Mechanism
The Frank-Starling mechanism is a physiologic description of the increased pumping action of the heart with increased filling. A larger preload results in increased contraction necessary to eject the added ventricular volume, resulting in a larger SV and similar EF. Reduced ventricular filling, as in hypovolemia, results in reduced SV. Small increases in preload may have dramatic effects (“volume responsiveness”) on SV and CO ( Fig. 5.2 ). At higher points on the curve, little additional benefit is derived from increases in preload.

Causes of Low Preload
Causes of low preload include hypovolemia and venodilation. Hypovolemia may result from hemorrhage or fluid losses. Venodilation occurs with general anesthesia and may be even more prominent in the presence of neuraxial anesthesia (also see Chapter 17 ). Additional causes of decreased preload include tension pneumothorax and pericardial tamponade, which prevent ventricular filling due to increased pressure around the heart, even though blood volume and filling pressures are adequate. Such conditions may manifest with systolic pressure variation (SPV), which describes changes in SBP with tidal breathing or ventilation that can be observed on an arterial blood pressure tracing. The extreme form of this is pulsus paradoxus, a pulse that changes markedly during tidal breathing. In the setting of normal or increased CVP, the presence of cardiac tamponade may exist. Pulse pressure variation ((PP peak – PP nadir )/PP average ) is analogous to SPV but requires computer calculation. Both high SPV and pulse pressure variation (PPV) are also useful in identifying hypovolemia, and are more sensitive and specific indicators of intravascular volume responsiveness than filling pressures.
Pathologic problems on the right side of the heart may prevent filling of the left ventricle. Pulmonary embolism and other causes of pulmonary hypertension prevent the right side of the heart from pumping a sufficient volume to fill the left side of the heart. The interventricular septum may be shifted, further constricting filling of the left side of the heart.
Contractility
Contractility, or the inotropic state of the heart, is a measure of the force of contraction independent of loading conditions (preload or afterload). It can be measured for research purposes by the rate at which pressure develops in the cardiac ventricles (dP/dT) or by systolic pressure-volume relationships ( Fig. 5.3 ). Decreased myocardial contractility may be a cause of hypotension ( Box 5.1 ).

Myocardial ischemia
Anesthetic drugs
Cardiomyopathy
Previous myocardial infarction
Valvular heart disease (decreased stroke volume independent of preload)
Afterload
Afterload is the resistance to ejection of blood from the left ventricle with each contraction. Clinically, afterload is largely determined by SVR. When SVR is increased, the heart does not empty as completely, resulting in a lower SV, EF, and CO (see Fig. 5.2 ). High SVR also increases cardiac filling pressures. Low SVR improves SV and increases CO such that a low SVR is often associated with a higher CO ( Fig. 5.4 ).

Low SVR decreases cardiac filling pressures. This finding may suggest that preload rather than afterload is the cause of hypotension. Low SVR allows more extensive emptying and a lower end-systolic volume (ESV), one of the hallmarks of low SVR on TEE. With the same venous return, the heart does not fill to the same EDV, resulting in lower left ventricular filling pressures (see Fig. 5.4 ). A similar process occurs when the SVR is increased. Such stress-induced increases in cardiac filling pressures are more pronounced in patients with poor cardiac function.
Cardiac Reflexes
The cardiovascular regulatory system consists of peripheral and central receptor systems that can detect various physiologic states, a central “integratory” system in the brainstem, and neurohumoral output to the heart and vascular system. A clinical understanding of cardiac reflexes is based on the concept that the cardiovascular system in the brainstem integrates the signal and provides a response through the autonomic nervous system.
Autonomic Nervous System
The heart and vascular systems are controlled by the autonomic nervous system. Sympathetic and parasympathetic efferents innervate the sinoatrial and atrioventricular nodes. Sympathetic nervous system stimulation increases HR through activation of β 1 -adrenergic receptors. Parasympathetic nervous system stimulation can profoundly slow HR through stimulation of muscarinic acetylcholine receptors in the sinoatrial and atrioventricular nodes, whereas parasympathetic nervous system suppression contributes to increased HR. Conduction through the atrioventricular node is increased and decreased by sympathetic and parasympathetic nervous system innervation, respectively. Sympathetic nervous system stimulation increases myocardial contractility. Parasympathetic nervous system stimulation may decrease myocardial contractility slightly, but it has its major effect through decreasing HR.
Baroreceptors
Baroreceptors in the carotid sinus and aortic arch are activated by increased systemic blood pressure that stimulates stretch receptors to send signals through the vagus and glossopharyngeal nerves to the central nervous system. The sensitivity of baroreceptors to systemic blood pressure changes varies and is significantly altered by long-standing essential hypertension. A typical response to acute hypertension is increased parasympathetic nervous system stimulation that decreases HR. Vagal stimulation and decreases in sympathetic nervous system activity also decrease myocardial contractility and cause reflex vasodilatation. This carotid sinus reflex can be used therapeutically to produce vagal stimulation that may be an effective treatment for supraventricular tachycardia.
The atria and ventricles are innervated by a variety of sympathetic and parasympathetic receptor systems. Atrial stretch (i.e., Bainbridge reflex) can increase HR, which may help match CO to venous return.
Stimulation of the chemoreceptors in the carotid sinus has respiratory and cardiovascular effects. Arterial hypoxemia results in sympathetic nervous system stimulation, although more profound and prolonged arterial hypoxemia can result in bradycardia, possibly through central mechanisms. A variety of other reflexes include bradycardia with ocular pressure (i.e., oculocardiac reflex) and bradycardia with stretch of abdominal viscera. The Cushing reflex includes bradycardia in response to increased intracranial pressure.
Many anesthetics blunt cardiac reflexes in a dose-dependent fashion, with the result that sympathetic nervous system responses to hypotension are reduced. The blunting of such reflexes represents an additional mechanism by which anesthetic drugs contribute to hypotension.
Coronary Blood Flow
The coronary circulation is unique in that a larger percentage of oxygen is extracted by the heart than in any other vascular bed, up to 60% to 70%, compared with the 25% extraction for the body as a whole. The consequence of this physiology is that the heart cannot increase oxygen extraction as a reserve mechanism. In cases of threatened oxygen supply, vasodilatation to increase blood flow is the primary compensatory mechanism of the heart.
Coronary reserve is the ability of the coronary circulation to increase flow more than the baseline state. Endogenous regulators of coronary blood flow include adenosine, nitric oxide, and adrenergic stimulation. With coronary artery stenosis, compensatory vasodilatation downstream can maintain coronary blood flow until about 90% stenosis, when coronary reserve begins to become exhausted.
The perfusion pressure of a vascular bed is usually calculated as the difference between MAP and venous pressure. Instantaneous flow through the coronary arteries varies throughout the cardiac cycle, peaking during systole. The heart is fundamentally different from other organs, because the myocardial wall tension developed during systole can completely stop blood flow in the subendocardium. The left ventricle is therefore perfused predominantly during diastole. The end-diastolic pressure in the left ventricle (LVEDP) may exceed CVP and represents the effective downstream pressure. Perfusion pressure to most of the left ventricle is therefore DBP minus LVEDP. The right ventricle, with its lower intramural pressure, is perfused during diastole and systole.
Pulmonary Circulation
The pulmonary circulation includes the right ventricle, pulmonary arteries, pulmonary capillary bed, and pulmonary veins, ending in the left atrium. The bronchial circulation supplies nutrients to lung tissue and empties into the pulmonary veins and left atrium. The pulmonary circulation differs substantially from the systemic circulation in its regulation, normal pressures ( Table 5.1 ), and responses to drugs. Use of a PA catheter to measure pressures in the pulmonary circulation requires a fundamental understanding of their normal values and their meaning. Pulmonary hypertension has idiopathic causes and may accompany several common diseases (e.g., cirrhosis of the liver, sleep apnea). It is associated with significant anesthetic-related morbidity and mortality rates.
Value | CVP (mm Hg) | PAS (mm Hg) | PAD (mm Hg) | PAM (mm Hg) | PCWP (mm Hg) |
---|---|---|---|---|---|
Normal | 2-8 | 15-30 | 4-12 | 9-16 | 4-12 |
High | >12 | >30 | >12 | >25 | >12 |
Pathologic | >18 | >40 | >20 | >35 | >20 |
Pulmonary Artery Pressure
Pulmonary artery pressure (PAP) is much lower than systemic pressure because of low pulmonary vascular resistance (PVR). Like the systemic circulation, the pulmonary circulation accepts the entire CO and must adapt its resistance to meet different conditions.
Pulmonary Vascular Resistance
Determinants of PVR are different from SVR in the systemic circulation. During blood flow through the pulmonary circulation, resistance is thought to occur in the larger vessels, small arteries, and capillary bed. Vessels within the alveoli and the extra-alveolar vessels respond differently to forces within the lung.
The most useful physiologic model for describing changes in the pulmonary circulation is the distention of capillaries and the recruitment of new capillaries. The distention and recruitment of capillaries explain the changes in PVR in a variety of circumstances. Increased PAP causes distention and recruitment of capillaries, increasing the cross-sectional area and decreasing PVR. Increased CO also decreases PVR through distention and recruitment. The reciprocal changes between CO and PVR maintain pulmonary pressures fairly constant over a wide range of CO values.
Lung volumes have different effects on intra-alveolar and extra-alveolar vessels. With large lung volumes, intra-alveolar vessels can be compressed, whereas extra-alveolar vessels have lower resistance. The opposite is true at small lung volumes. Therefore, higher PVR occurs at large and small lung volumes. Increased PVR at small lung volumes helps to divert blood flow from collapsed alveoli, such as during one-lung ventilation.
Sympathetic nervous system stimulation can cause pulmonary vasoconstriction, but the effect is not large, in contrast to the systemic circulation, in which neurohumoral influence is the primary regulator of vascular tone. The pulmonary circulation has therefore been very difficult to treat with drugs. Nitric oxide is an important regulator of vascular tone and can be given by inhalation. Prostaglandins and phosphodiesterase inhibitors (e.g., sildenafil) are pulmonary vasodilators, but the pharmacologic responses that can be achieved in pulmonary hypertension are limited.
Hypoxic Pulmonary Vasoconstriction
Hypoxic pulmonary vasoconstriction (HPV) is the pulmonary vascular response to a low alveolar oxygen partial pressure (P ao 2 ). In many patients, HPV is an important adaptive response that improves gas exchange by diverting blood away from poorly ventilated areas, decreasing shunt fraction. Normal regions of the lung can easily accommodate the additional blood flow without increasing PAP. Global alveolar hypoxia, such as occurs with apnea or at high altitude, can cause significant HPV and increased PAP.
Anesthetic drugs such as the potent inhaled anesthetics can impair HPV, whereas commonly used intravenous drugs, such as propofol and opioids, demonstrate no inhibition of HPV. During surgical procedures requiring one-lung ventilation, HPV may play a role in the resolution of hypoxemia, although many other factors are also important, including acid-base status, CO, development of atelectasis, and concomitant drug administration.
Pulmonary Emboli
Pulmonary emboli obstruct blood vessels, increasing the overall resistance to blood through the pulmonary vascular system. Common forms of emboli are blood clots and air, but they also include amniotic fluid, carbon dioxide, and fat emboli.
Arteriolar Thickening
Arteriolar thickening occurs in several clinical circumstances. It is associated with certain types of long-standing congenital heart disease. Primary pulmonary hypertension is an idiopathic disease associated with arteriolar hyperplasia. Similar changes are associated with cirrhosis of the liver (i.e., portopulmonary hypertension).
Zones of the Lung
A useful concept in pulmonary hemodynamics is West’s zones of the lung. Gravity determines the way pressures change in the vascular system relative to the measurement at the level of the heart. These differences are small compared with arterial pressures, but for venous pressure and PAP, these differences are clinically significant. Every 20 cm of change in height produces a 15-mm Hg pressure difference. This can create significant positional differences in PAP that affect blood flow in the lung in various positions, such as upright and lateral positions.
In zone 1, airway pressures exceed PAP and pulmonary venous pressures. Zone 1 therefore has no blood flow despite ventilation. Normally, zone 1 does not exist, but with positive-pressure ventilation or low PAP, as may occur under anesthesia or with blood loss, zone 1 may develop. In zone 2, airway pressure is more than pulmonary venous pressure, but it is not more than PAP. In zone 2, flow is proportional to the difference between PAP and airway pressure. In zone 3, PAP and venous pressure exceed airway pressure, and a normal blood flow pattern results (i.e., flow is proportional to the difference between PAP and venous pressure). Position can also be used therapeutically to decrease blood flow to abnormal areas of the lung, such as unilateral pneumonia, and thereby improve gas exchange. Blood flow through the collapsed lung during one-lung ventilation is also reduced by this physiologic effect.
Pulmonary Edema
Intravascular fluid balance in the lung depends on hydrostatic driving forces. Excessive pulmonary capillary pressures cause fluid to leak into the interstitium and then into alveoli. Although the pulmonary lymphatic system is very effective in clearing fluid, it can be overwhelmed. Hydrostatic pulmonary edema is expected with high left ventricular filling pressures. Pulmonary edema occurs as PCWP exceeds 20 mm Hg, although patients may tolerate even higher pressures if these pressures persist chronically. Pulmonary edema can also occur with “capillary leak” from lung injury, such as acid aspiration of gastric contents, sepsis, or blood transfusion.
Pulmonary Gas Exchange
Oxygen
Oxygen must pass from the environment to the tissues, where it is consumed during aerobic metabolism. Arterial hypoxemia is defined as a low partial pressure of oxygen in arterial blood (Pa o 2 ). An arbitrary definition of arterial hypoxemia (Pa o 2 < 60 mm Hg) is commonly used but not necessary. Occasionally, arterial hypoxemia is used to describe a Pa o 2 that is low relative to what might be expected based on the inspired oxygen concentration (F io 2 ). Arterial hypoxemia (which reflects pulmonary gas exchange) is distinguished from hypoxia, a more general term including tissue hypoxia, which also reflects circulatory factors.
Mild and even moderate arterial hypoxemia (e.g., at high altitude) can be well tolerated and is not usually associated with substantial injury or adverse outcomes. Anoxia, a nearly complete lack of oxygen, is potentially fatal and is often associated with permanent neurologic injury, depending on its duration. Arterial hypoxemia is most significant when anoxia is threatened, such as with apnea, and the difference between the two may be less than 1 minute.
Measurements of Oxygenation
Measurements of arterial blood oxygen levels include Pa o 2 , oxyhemoglobin saturation (Sa o 2 ), and arterial oxygen content (Ca o 2 ). Pa o 2 and Sa o 2 are related through the oxyhemoglobin dissociation curve ( Fig. 5.5 ). Understanding the oxyhemoglobin dissociation curve is facilitated by the ability to measure continuous oxyhemoglobin saturation with pulse oximetry (Sp o 2 ) and measurement of Pa o 2 with arterial blood gas analysis.

Oxyhemoglobin Dissociation Curve
Rightward and leftward shifts of the oxyhemoglobin dissociation curve provide significant homeostatic adaptations to changing oxygen availability. P 50 , the P o 2 at which hemoglobin is 50% saturated with oxygen, is a measurement of the position of the oxyhemoglobin dissociation curve (see Fig. 5.5 , Table 5.2 ). The normal P 50 value of adult hemoglobin is 26.8 mm Hg. Other points on the curve, such as the normal venous point and points for 80% and 90% oxygen saturations may also be clinically useful.
Left Shift | Right Shift |
---|---|
(P 50 < 26.8 mm Hg) | (P 50 > 26.8 mm Hg) |
Alkalosis | Acidosis |
Hypothermia | Hyperthermia |
Decreased 2,3-diphosphoglycerate (stored blood) | Increased 2,3-diphosphoglycerate (chronic arterial hypoxemia or anemia) |
A rightward shift causes little change in conditions for loading oxygen (essentially the same Sa o 2 at P o 2 of 100 mm Hg), but it allows larger amounts of oxygen to dissociate from hemoglobin in the tissues. This improves tissue oxygenation. Carbon dioxide and metabolic acid shift the oxyhemoglobin dissociation curve rightward, whereas alkalosis shifts it leftward. Fetal hemoglobin is left shifted, an adaptation uniquely suited to placental physiology. Oxygen in arterial blood is bound to hemoglobin and dissolved in the plasma. The blood oxygen content is the sum of the two forms. Although amounts of dissolved oxygen are fairly trivial at normal P o 2 levels, at high F io 2 dissolved oxygen can be physiologically and clinically important. Although under normal conditions only a fraction (25%) of the oxygen on hemoglobin is used, all of the added dissolved oxygen added while giving supplemental oxygen can be used.
Arterial Oxygen Content
Ca o 2 is calculated based on Sa o 2 and partial pressure plus the hemoglobin concentration ( Fig. 5.6 ):
CaO2=SaO2(Hb×1.39)+0.003(PaO2)
CaO2=SaO2(Hb×1.39)+0.003(PaO2)
CaO2=SaO2(Hb×1.39)+0.003(PaO2)

In the equation, Hb is the hemoglobin level, 1.39 is the capacity of hemoglobin for oxygen (1.39 mL of O 2 /g of Hb fully saturated), and 0.003 mL O 2 /dL/mm Hg is the solubility of oxygen. For example, if Hb = 15 g/dL and Pa o 2 = 100 mm Hg, resulting in nearly 100% saturation, the value of Ca o 2 is calculated as follows:
CaO2=1.00(15×1.39)+100(0.003)=20.85+0.3=21.15mL/dL
CaO2=1.00(15×1.39)+100(0.003)=20.85+0.3=21.15mL/dL
CaO2=1.00(15×1.39)+100(0.003)=20.85+0.3=21.15mL/dL
Dissolved oxygen can continue to provide additional Ca o 2 , which can be clinically significant, with F io 2 of 1.0 and with hyperbaric oxygen. The oxygen cascade depicts the passage of oxygen from the atmosphere to the tissues ( Fig. 5.7 ).

V˙/Q˙
˙V/˙Q
V ˙ / Q ˙
) mismatch. Oxygen consumption then reduces P o 2 to tissue levels (about 40 mm Hg).
Multiwavelength Pulse Oximetry
Complete measurement of oxygen parameters are derived not just from analysis of arterial blood gases (Pa o 2 ) but also from multiwavelength pulse oximetry. Oximetry provides measurement of methemoglobin (MetHb) and carboxyhemoglobin (COHb). Most blood gas machines are now combined with oximeters so that the Sa o 2 provided is a true measured value, not calculated. This is called the functional saturation , which is the percent oxyhemoglobin saturation relative to hemoglobin available to bind oxygen. The fractional saturation is relative to all hemoglobin. Therefore, fractional saturation is the functional saturation minus MetHb and COHb. Newer pulse oximeters can now also measure MetHb and COHb.
Determinants of Alveolar Oxygen Partial Pressure
The alveolar gas equation describes transfer of oxygen from the environment into the alveoli:
PAO2=FIO2×(PB−PH2O)−PCO2/RQ
PAO2=FIO2×(PB−PH2O)−PCO2/RQ
PAO2=FIO2×(PB−PH2O)−PCO2/RQ
PAO2=1.0(760−47)−40/0.8=713−50=663mmHg
PAO2=1.0(760−47)−40/0.8=713−50=663mmHg
PAO2=1.0(760−47)−40/0.8=713−50=663mmHg
The alveolar gas equation describes the way in which inspired oxygen and ventilation determine Pa o 2 . It also describes the way in which supplemental oxygen improves oxygenation. One clinical consequence of this relationship is that supplemental oxygen can easily compensate for the adverse effects of hypoventilation ( Fig. 5.8 ).

Low barometric pressure is a cause of arterial hypoxemia at high altitude. Modern anesthesia machines have safety mechanisms to prevent delivery of hypoxic gas mixtures. Nevertheless, death from delivery of gases other than oxygen is still occasionally reported because of errors in pipe connections made during construction or remodeling of operating rooms. Current anesthesia machines have multiple safety features to prevent delivery of hypoxic gas mixtures. Delivery of an inadequate F io 2 may occur when oxygen tanks run out or with failure to recognize accidental disconnection of a self-inflating bag (Ambu) from its oxygen source.
Apnea is an important cause of arterial hypoxemia, and storage of oxygen in the lung is of prime importance in delaying the appearance of arterial hypoxemia in humans. Storage of oxygen on hemoglobin is secondary, because use of this oxygen requires significant oxyhemoglobin desaturation. In contrast to voluntary breath-holding, apnea during anesthesia or sedation occurs at functional residual capacity (FRC). This substantially reduces the time to oxyhemoglobin desaturation compared with a breath-hold at total lung capacity.
The time can be estimated for Sa o 2 to reach 90% when the FRC is 2.5 L and the Pa o 2 is 100 mm Hg. Normal oxygen consumption is about 300 mL/min, although this is somewhat lower during anesthesia. It would take only about 30 seconds under these room air conditions to develop arterial hypoxemia. After breathing 100% oxygen, it might take 7 minutes to reach an Sa o 2 of 90%. In reality, the time it takes to develop arterial hypoxemia after breathing 100% oxygen varies. Desaturation begins when sufficient numbers of alveoli have collapsed and intrapulmonary shunt develops, not simply when oxygen stores have become exhausted. In particular, obese patients develop arterial hypoxemia with apnea substantially faster than lean patients.
Venous Admixture
Venous admixture describes physiologic causes of arterial hypoxemia for which P ao 2 is normal. The alveolar-to-arterial oxygen (A-a) gradient reflects venous admixture. Normal A-a gradients are 5 to 10 mm Hg, but they increase with age. For example, if the arterial P o 2 while breathing 100% oxygen were measured as 310 mm Hg, the A-a gradient can be calculated from the previous example.
A-agradient=PAO2−PaO2=663mmHg−310mmHg=353mmHg
A-agradient=PAO2−PaO2=663mmHg−310mmHg=353mmHg
A-agradient=PAO2−PaO2=663mmHg−310mmHg=353mmHg
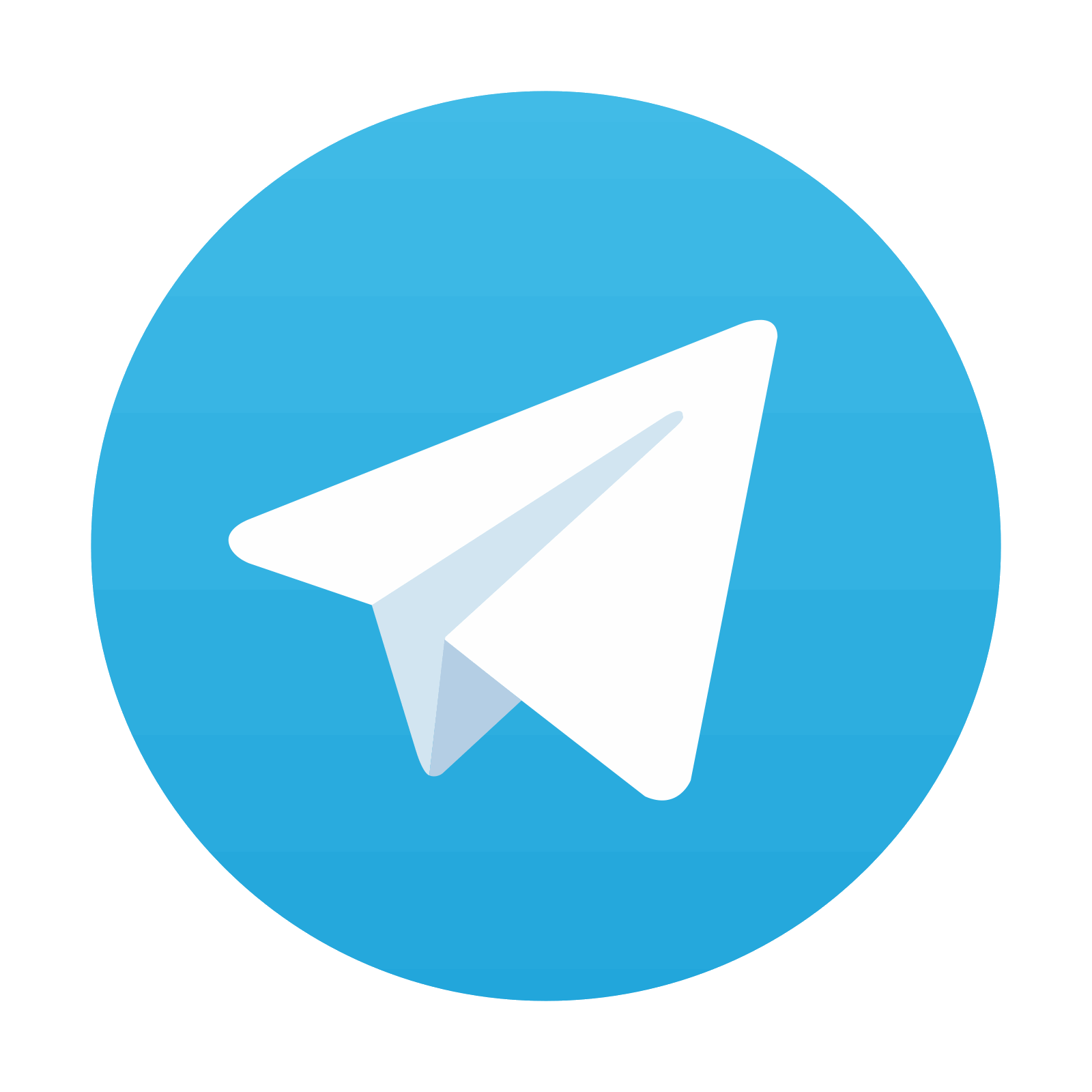
Stay updated, free articles. Join our Telegram channel
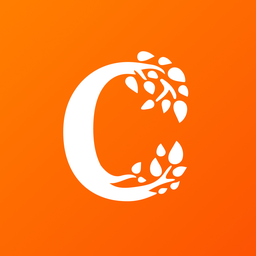
Full access? Get Clinical Tree
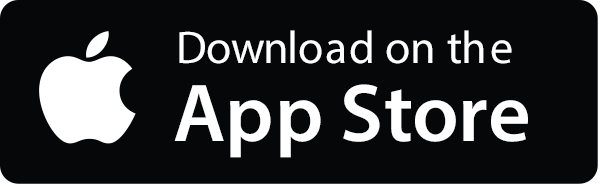
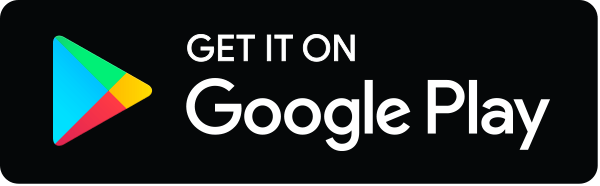
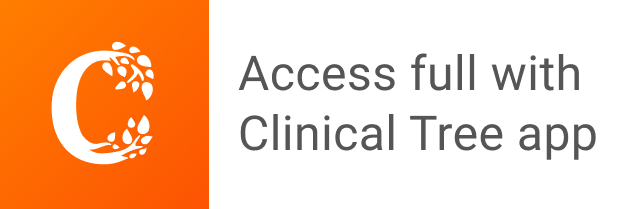