Introduction
Problems of clinical importance often are first noticed anecdotally, then more carefully documented and studied in order to define the problem and note associations with other aspects of the environment or individual. In turn, these associations form hypotheses for causation and mitigation. The clinical problem, perioperative neurocognitive disorders (PND), is clearly associated with anesthesia, surgery, and hospitalization, but these environmental influences are so exceedingly complex that mechanistic hypotheses are numerous and hopelessly intertwined. For example, anesthesia and surgery almost always co-exist, as well as a host of different drugs including, but certainly not limited to, anesthetics (both local and general), sedatives, neuromuscular relaxants and their reversal drugs, antiemetics, antibiotics, opioids, and laxatives. Pain, sleep disorders, delirium, nutritional lapses, infection, and emotional stress are common postoperative events. Even though the general anesthetic is often implicated in subsequent disorders of cognition, each of the aforementioned factors could also play a role. Sorting these variables out with clinical studies is nearly impossible as many simply must co-exist because their combination constitutes optimal health care. Finally, in addition to the complexity of the clinical arena and perioperative care, the patients themselves bring along a host of individual factors, including their unique genetics, habits, and comorbidities, including baseline cognitive impairment, frailty, and cardiovascular disease. Thus, investigators have turned to simplified animal models to sort out the factors, one at a time, to establish mechanisms and effect magnitude in order to propose mitigation strategies (see Chapter 16). In this chapter, we will present and discuss the various animal models that investigators have used for PND studies, including anesthesia alone, anesthesia and surgery, and various preexisting vulnerabilities with, or without, anesthesia and surgery. Almost all of the work has been performed in rodents, a compromise between translatability, expense, and time. The benefits and limitations of using animals in PND studies are addressed, along with a series of recommendations for the future.
Animal Models: General Considerations
Anesthesia
The possibility that the anesthetic contributed to PND was first posed in a study using elderly rats.[1] This was followed by a host of animal studies, almost all of which were in healthy adult wild-type rats or mice, exposed to a general anesthetic for several hours, usually breathing spontaneously, allowed to emerge, and then subjected to various behavioral testing at intervals after the anesthetic, typically up to a week and rarely more than a month. With the introduction of vulnerable animals and surgery to the studies, it has become clear that the anesthetic may have modulatory effects on outcomes but that the vulnerability of the animal prior to surgery and the inflammatory trauma as a result of surgery play far more significant roles in determining outcomes.
Class of Anesthetic/Route of Administration
The relative ease of delivering anesthesia by inhalation makes these drugs the most commonly used clinically, and also the most commonly used in animal studies. The potency of all the currently used gases (isoflurane, desflurane, and sevoflurane) is very similar in all mammals, making studies in the rodent of reasonable relevance. The use of injectable anesthetics in rodents, on the other hand, is more problematic. In humans, injectable drugs are almost always given intravenously. While this can also be performed in rodents using the tail vein approach, many investigators instead use the intraperitoneal (IP) approach. A commonly used route of administration for a wide variety of medications in small animals, the IP approach is never used in humans. While potentially relevant to clinical practice, IP administration has different pharmacokinetics and possibly different inflammatory and immunological implications. Oral administration of drugs is a common route for rodents, either by gavage or adding to drinking water, but a rarely used route for general anesthetics.
Physiological Control and Monitoring
General anesthetics have many side effects, some of which are potentially lethal, and they occur at drug concentrations very close to those required for the primary effect, anesthesia. These side effects include cardiovascular and respiratory depression and thermal dysregulation, all of which could independently injure (or protect) the brain and contribute to any subsequent cognitive disorders. In clinical anesthesia, these side effects are carefully monitored and mitigated where necessary. This is almost never true in rodent studies, as it is very difficult to control the ventilation or monitor blood gases in animals weighing only 30 grams. Obtaining blood for the measurement of blood gases or chemistry, for example, is often a terminal procedure because of the volume required. Small, rapid-response pulse oximeters that can provide both heart rate and oxygen saturation are becoming available, but these cannot provide a measure of CO2 or pH, both measures of the adequacy of ventilation. Blood pressure and cardiac output are also difficult to monitor. Unexpected mortality, a good indicator of significant side effects, is rarely reported. Thus, rodent anesthesia is often poorly monitored and therefore significantly contaminated with physiological derangements that can contribute to, or cause, cognitive dysfunction and confound data interpretation. Also the percent oxygen used with an inhaled anesthetic needs to be determined for aged rodents.
Scaling
An issue of considerable importance, but also of ambiguity, is anesthetic exposure scaling. For example, is a 6 hour anesthetic exposure in an animal whose entire life-span is 2 years equivalent to a 250 hour anesthetic in a human that lives 80 years? Is rodent cognitive performance at 1 week postexposure analogous to that in a human at 1 year? At the most fundamental level, atoms, molecules, and cells move and respond with the same kinetics in both human and rodent, but some organs function at much greater speed (e.g., the heart). On the other hand, a rodent’s circadian rhythm is analogous to that of a human and, as mentioned above, anesthetic potency is almost identical. Therefore, it is simply not clear whether adjustments to exposure lengths or recovery periods need to be made to account for life-span differences. Currently, most investigators do not.
Superimposed Stress and Inflammation
Surgery
Anesthesia is rarely given to patients in the absence of a procedure that would normally cause pain and suffering. Such a procedure could itself cause cognitive disturbance, or may alter the effect of the anesthetic. Thus, investigators have begun to include a surgical procedure in their models to more closely simulate what happens to patients. Importantly, it has become clear in recent years that the surgical injury produces activation of the animal’s innate immune system, inducing up-regulation of many inflammatory mediators in the periphery and in the brain in animal models. Moreover, a number of endogenous molecules, called alarmins, are released from damaged cells during injuries such as fractures and many of these have consequences for cognition.[2–7] The surgical procedures used in modeling this have varied, and each has their strengths and limitations, especially when applied to healthy adult animals. The remainder of this chapter will refer to studies that included surgery or other systemic inflammatory insults primarily in aged animals.
Orthopedic. Because orthopedic surgery is exceedingly common in the geriatric populations, some investigators have developed orthopedic models though thus far they have not been used in aged animals. Most commonly, the tibia is fractured under anesthesia in a mouse, and then repaired with an intramedullary rod, simulating a common hip fracture. The advantages are the consistency and relevance, but the disadvantage is that pain and tissue damage may limit the animal’s mobility. Cognition and motor ability can be difficult to separate in conventional rodent maze tasks only a few days after surgery, while other cognitive tests, such as fear conditioning, may not be similarly affected.
Abdominal. Abdominal procedures are also common in the elderly population, including colectomy, herniorrhaphy, small bowel resection, prostatectomy, etc. In the rodent, several aseptic abdominal procedures have been reported in aged wild-type rodents and Alzheimer transgenic models, ranging from a simple laparotomy[2, 4, 8] to partial hepatectomy,[9, 10] splenectomy,[9, 11] nephrectomy[12] to cecal ligation and excision (without puncture).[13, 14] As in the orthopedic procedures, postoperative pain may limit movement-dependent behavioral assays, especially if tested soon after the surgery. Analgesics, however, introduce new confounders that have to date not been considered to influence outcome.
Models of Systemic Inflammation (Sepsis and LPS)
Surgery constitutes a significant inflammatory trauma and shares many features with the systemic inflammatory responses observed during systemic infection and sepsis. Indeed a number of endogenous molecules released during tissue injury (alarmins such as high mobility group box 1, HMGB1) activate the same immune system receptors as those activated by gram-negative bacterial endotoxin (lipopolysaccharide, LPS) and lead to release of a similar repertoire of inflammatory mediators. As such, studies of systemic inflammation induced by LPS or sepsis can and have[15] provided information on cognitive impairments relevant to PND.
Moreover, not all surgery is clean, and sepsis syndrome among the elderly receiving operative care is not uncommon. Sepsis is also associated with significant morbidity and mortality, as well as subsequent cognitive problems in humans, especially the elderly,[16, 17] and in rodent sepsis survivors.[18–20] One model of sepsis in the rodent consists of ligating and puncturing the cecum (CLP), to lead to abscess formation and fecal contamination of the abdomen. This “CLP” surgery leads to a pronounced sepsis syndrome, the survival from which depends on a number of operative (number of punctures) and postoperative (fluids, antibiotics, warming) manipulations. Mortality can be very high, and thus this is rightly considered a model of septic shock rather than a model of surgery. Although an extreme one, it is not an irrelevant model of surgery but the microbial contribution to innate immune responses considerably outweighs the surgical/traumatic contribution. A limitation of this surgery can be the generation of a “survivor-effect,” whereby the animals/humans that do not die, either did not receive the same insult or become resistant in some way to the insult and its consequences, and go on to do as well or better than the controls.[21, 22]
In order to simplify the sepsis syndrome to the upstream initiators, some investigators administer purified LPS, either IV or IP, to induce a transient and robust systemic inflammation or “sickness behavior” with neuroinflammation and changes in cognition.[23–26] This procedure is done in the absence of anesthesia, which may be able to help tease out the effects of the anesthetic. Whether this sickness behavior constitutes delirium or PND is not clear, although it does not always produce cognitive impairment.[27] However, these challenges do activate innate immunity and neuroinflammation and overlap significantly with inflammatory activation occurring during aseptic surgery.
Animal Models of Preexisting Vulnerabilities
A general theme to this chapter on animals, as well as the design of clinical studies, is that the enrichment of the study population for vulnerabilities (age being one) enhances the probability of detecting relevant effects so that mitigation strategies can be tested (Table 6.1). Not at all patients and animals suffer cognitive disorders following anesthesia and surgery; there are clearly preexisting vulnerabilities that interact with the stress of anesthesia and surgery. This interaction could be very specific, so that knowing the vulnerability may provide clues as to the pathophysiology by which anesthesia and surgery produce acute cognitive dysfunction and possibly even accelerate decline. Age is a key vulnerability factor, as we mentioned above, but this may encompass multiple distinct risk factors. One key feature in the aged human population is the occurrence of cognitive impairment at baseline (i.e., preoperatively) and surgery in aged rodents can produce deficits that are exaggerated as compared to deficits produced in young healthy animals.
Animal Model | Preexisting Vulnerability | Event | Intervention | Post-op Cognitive Test/Time | Results of Cognitive Testing | Reference | |
---|---|---|---|---|---|---|---|
Anesthesia | Surgery | ||||||
Mice BALB/c | Age 23–25 mos | Ketamine and xylazine, i.p. | Laparotomy | – | MWM 24 hours | Not impaired: Surgery | [2] |
Rats Sprague-Dawley | Age 20–24 mos | Isoflurane | Partial hepatectomy | – | MWM 1, 3, 7 days | Impaired: Surgery | [42] |
Mice C57BL/6 | Age 14 mos | Chloral hydrate, i.p. | Partial splenectomy | Celastrol, i.p. | MWM 1, 3, 7 days | Impaired: Surgery Not impaired: Surgery + Celastrol | [9] |
Mice C57BL/6 | Age 16 mos | Isoflurane | Partial hepatectomy | – | MWM 31–35 days | Improved cognition: Surgery | [43] |
Rats F344/BN | Age 24 mos | Halothane | Laparotomy | hIL-1RA (intracisternal /i.p.) | FC days 4, 12 | Impaired: Surgery + hIL-1RA (i.p. injection) Not impaired: Surgery + hIL-1RA (intracisternal injection) | [4] |
Rats Sprague-Dawley | Age 22–23 mos | Isoflurane | Partial Splenectomy | – | MWM 1, 3, 7 days | Impaired: Surgery | [44] |
Rats LCR, HCR | Metabolic syndrome, Age 4–5 mos | Isoflurane | Tibial fracture with fixation | – | FC 1–7 days MWM 5 mos | Impaired: Surgery + LCR > Surgery + HCR | [45] |
Rats Wistar | Age 18–20 mos | Sevoflurane | Laparotomy + upper mesenteric artery clamped | – | MWM, Y-Maze, OF, EPM, FC days 7, 35 | Impaired: Surgery (affective behavior only) Not impaired: Surgery (cognition) | [29] |
Rats Sprague-Dawley | Age 20 mos | Isoflurane | Partial hepatectomy | Ulinastatin, i.v. | MWM 3, 7, 15 days | Impaired: Surgery Not impaired: Surgery + Ulinastatin | [46] |
Mice C57BL/6 | Age 14 mos | Isoflurane | Partial hepatectomy | – | Y-maze 1, 3, 7 | Impaired: Surgery | [47] |
Rats LCR, HCR | Metabolic syndrome, Age 4 mos | Isoflurane | Tibial fracture with fixation | – | FC 3 days | Impaired: Surgery + LCR > Surgery + HCR | [48] |
Mice 3xTgAD (App/tau/PS1) | AD transgenic Age 5–11 mos | Desflurane | Abdominal (cecal ligation & excision) | – | MWM 2 weeks; 14 weeks | Impaired: Surgery | [13] |
Rats Sprague-Dawley | Age 22 mos | Fentanyl + droperidol, i.p. | Splenectomy | – | Y-maze 1, 3, 9 days | Impaired: Surgery | [11] |
Rats Fischer 344 | Age 20 mos | Propofol Isoflurane | Right carotid exposure | – | BM FC 6–18 days | Impaired: Surgery + Propofol; Surgery + Isoflurane | [37] |
Rats Sprague-Dawley | Age 18–22 mos | Chloral hydrate | Splenectomy | – | MWM 1, 3, 5, 7 days | Impaired: Surgery | [49] |
Mice C57BL/6 | Age 19 mos | Sodium pentobarbital, i.p. | Partial hepatectomy | Minocycline, i.p. | MWM 31–35 days | Impaired: Surgery Not impaired: Surgery + Minocycline | [50] |
Rats Wistar | Age 24–25 mos | Isoflurane | Laparotomy (incision only) | Ketoprofen, SQ | RAM 3–14 days | Impaired: Surgery Not impaired: Surgery + Ketoprofen, Surgery + Morphine | [51] |
Rats Sprague-Dawley | Age 18 mos | Sevoflurane | Partial hepatectomy | – | MWM 1, 3, 7 days | Impaired: Surgery | [10] |
Rats Sprague-Dawley | Age 20 mos | Isoflurane | Laparotomy | Candesartan | MWM 2–7 days | Impaired: Surgery Not impaired: Surgery + Candesartan | [52] |
Mice ADTg (APP/PS1) C57BL/6J | AD transgenic Age 9 mos, ADTg 18 mos, WT | Bupivacaine | Laparotomy (incision only) | – | FC 24h, 72h, 7, 30, 60 days MWM 1–7 days | Impaired: Surgery ADTg; Surgery WT | [8] |
Mice C57BL/6 | Age 12 mos | Ketamine and xylazine, i.p. | Nephrectomy | RNase A, SQ | MWM 1, 3, 7 days | Impaired: Surgery Not impaired: Surgery; Surgery + RNase A | [12] |
Rats LCR, HCR | Metabolic syndrome, Age 4 mos | Isoflurane | Tibial fracture with fixation | – | FC 1week MWM 3 mos | Impaired: Surgery (LCR + HCR) 1 week Surgery LCR 3 mos Not impaired: HCR 3 mos; Anesthesia only | [54] |
Rats Wistar | Age 25 mos | Sevoflurane | Laparotomy + upper mesenteric artery clamped | – | MWM, NOR OF 9–14 days | Impaired: Surgery | [34] |
Rats Wistar | Age 18 mos, Prior infection | Sevoflurane | Laparotomy + upper mesenteric artery clamped | – | OF NOR MWM 9–14 days | Impaired: Surgery + prior infection | [54] |
Mice C57BL/6 | Age 16 mos | Isoflurane Pentobarbital sodium (ICV implants) | Laparotomy ICV cannula implantation | SAHA, ICV injection | OF FC STM LTM 5–7 days | Impaired: Surgery Not impaired: Surgery + SAHA | [55] |
Rats Wistar | Age 24–25 mos | Isoflurane | Laparotomy | Environmental enrichment (PPE) | NOR 7 days | Impaired: Surgery Not impaired: Surgery + PPE | [56] |
Rats Sprague-Dawley | Age 18–20 mos | Isoflurane | Partial hepatectomy | – | Y-maze 1–2 days | Impaired: Surgery | [57] |
Rats Sprague-Dawley | Age 20 mos | Sevoflurane | Partial hepatectomy | – | MWM 8–12 days | Impaired: Surgery | [58] |
Rats Sprague-Dawley | Age 16 mos | Isoflurane | Splenectomy | Lithium, i.p. | MWM 1 day | Impaired: Surgery Not impaired: Surgery + Lithium | [33] |
Rats Sprague-Dawley | Age 24 mos | Pelltobarbitalum Natricum | Laparotomy | R‑7050, intracisternal injection | MWM 1, 3, 5 days | Impaired: Surgery Not impaired: Surgery + R‑7050 | [59] |
Mice BALB/c | Age 20–22 mos | Isoflurane | Splenectomy | Dexmedetomidine, i.p. | Y-maze 1, 3 days | Impaired: Surgery Not impaired: Surgery + Dexmedetomidine | [38] |
Mice C57BL/6 | Age 16 mos | Isoflurane | Laparotomy | Apocynin | OF FC 6–7 days | Impaired: Surgery Not impaired: Surgery + Apocynin | [60] |
Mice C57BL/6 | Age 14 mos | Sodium pentobarbital, i.p. | Partial hepatectomy ICV cannula implantation | IL17A antibody, ICV injection | MWM 1, 3, 7 days T-maze 1, 3, 7 days | Impaired: Surgery Not impaired: Surgery + IL17A antibody | [61] |
Mice C57BL/6 | Age 20 mos | Chloral hydrate, i.p. | Laparotomy | Mifepristone Roscovitine | MWM 5–13 days EPM 5 days | Impaired: Surgery Not impaired: Surgery + Roscovitine; Surgery + Mifepristone | [62] |
Mice C57BL/6 | Age 18 mos | Isoflurane | Appendectomy | – | MWM 3, 28 days | Impaired: Isoflurane; Surgery + Isoflurane | [63] |
Rats Wistar | Age 20–24 mos | Isoflurane | Laparotomy | Insulin, intranasal + pentobarbital anesthesia | NOR PO day 14 | Impaired: Surgery Not impaired: Surgery + intranasal insulin | [64] |
Rats Wistar | Age 24–25 mos | Isoflurane | Laparotomy | Pregabalin, i.p. (pre-op vs. PO) | NOR OF 14 days | Impaired: Surgery; Surgery + Pregabalin (PO) Not impaired: Surgery + Pregabalin (pre-op) | [65] |
Mice C57BL/6 | Age 12–14 mos | Chloral hydrate, i.p. | Laparotomy | Deferoxamine, i.p. | FC 3, 7, 14 days MWM 3, 7, 14 days | Impaired: Surgery Not impaired: Surgery + Deferoxamine | [66] |
Rats Sprague-Dawley | Age 18 mos | Chloral hydrate, i.p. | Laparotomy | Deferoxamine | MWM 1, 3, 7 days | Impaired: Surgery Not impaired: Surgery + Deferoxamine | [67] |
Rats Sprague-Dawley | Age 19–22 mos | Sevoflurane | Partial hepatectomy | anti-HMGB1 | BM 1–4 days | Impaired: Surgery Not impaired: Surgery + anti-HMGB1 | [68] |
Rats Wistar | Age 18 mos | Pentobarbital sodium, i.p. | Laparotomy + splenectomy | Dexmedetomidine | MWM 1, 3, 7 days | Impaired: Surgery Not impaired: Surgery + Dexmedetomidine | [69] |
Mice C57BL/6 | Age 20 mos | Isoflurane | Laparotomy | Berberine | OF FC 6–7 days | Impaired: Surgery Not impaired: Surgery + Berberine | [70] |
Rats LCR, HCR | Metabolic syndrome, Age 4 mos | Isoflurane | Tibial fracture with fixation | Preoperative exercise (PE) | FC 1week MWM 3 mos | Impaired: Surgery + PE (LCR + HCR) 1 week; Surgery LCR 3 mos Not impaired: Surgery + PE (LCR + HCR) 3 mos | [71] |
Rats Sprague-Dawley | Age 21–23 mos | Isoflurane | Partial hepatectomy | Electroacupuncture Minocycline, i.p. | MWM 3, 7 days | Impaired: Surgery Not impaired: Surgery + Electroacupuncture; Surgery + Minocycline | [72] |
Rats Sprague-Dawley | Age 18–20 mos | Isoflurane | Partial hepatectomy | IL-4, intracisternal injection | MWM 1, 3, 7 days | Impaired: Surgery Not impaired: Surgery + IL-4 | [73] |
Rats Sprague-Dawley | Age 18 mos | Chloral hydrate, i.p. | Partial hepatectomy | Electroacupuncture | MWM 1, 3, 7 days | Impaired: Surgery Not impaired: Surgery + Electroacupuncture | [74] |
Mice 3xTgAD (App/tau/PS1) | AD transgenic Age 11 mos | Propofol | Abdominal (cecal ligation & excision) | – | MWM 3 weeks; 15 weeks | Not impaired: Anesthesia + surgery | [14] |
Mice FAD5X | AD transgenic Age 5 mos | Isoflurane Desflurane | Laparotomy | Vitamin K2 | BM 3, 7, 14, 21, 28, 35, 42 days | Impaired: Surgery + Isoflurane (day 14) Not impaired: Surgery + Desflurane; Surgery + Isoflurane (all days except 14) | [75] |
Rats Sprague-Dawley | Age 18 mos | Isoflurane | Splenectomy | Nimodipine, Hypertonic Saline | MWM 1, 3, 7 days | Impaired: Surgery Not impaired: Surgery + Nimodipine + Hypertonic Saline | [76] |
Mice C57BL/6J | Age 14 mos | Bupivacaine, SQ | Laparotomy (incision only) | Edaravone | MWM 1–7 days T-maze 1, 3, 7 days | Impaired: Surgery Not impaired: Surgery + Edaravone | [77] |
Mice Kunming | Age 16 mos | Chloral hydrate, i.p. | Partial hepatectomy | Hydrogen-rich saline | MWM 8, 10, 14 days | Impaired: Surgery Not impaired: Surgery + Hydrogen-rich saline | [78] |
Mice C57BL/6 | Age 14 mos | Chloral hydrate, i.p. | Carotid artery exposure | Rolipram | MWM FC 3–12 days | Impaired: Surgery Not impaired: Surgery + Rolipram | [79] |
Rats Sprague-Dawley | Age 18 mos | Fentanyl + Droperidol, i.p. | Partial hepatectomy | Nicotine | MWM 1, 3 days | Impaired: Surgery Not impaired: Surgery + Nicotine | [80] |
Mice C57BL/6 | Age 12–14 mos | Isoflurane | Tibial fracture with fixation | – | OF FC 3 days | Impaired: Surgery | [81] |
Mice ADTg (APP/PS1) (ADTg-CypD KO) | AD transgenic, Cyclophilin D KO | Sevoflurane | Laparotomy (incision only) | – | FC 1–7 days | Impaired: Female ADTg Not impaired: Male ADTg; Female ADTg + CypD KO | [82] |
Mice C57BL/6 | Age 20 mos | Isoflurane | Laparotomy | Pioglitazone, GW9662 | NOR OF PAT 4–7 days | Impaired: Surgery; Surgery + GW9662 Not impaired: Surgery + Pioglitazone | [83] |
Rats Sprague-Dawley | Age 20–24 mos | Sevoflurane | Laparotomy | Compound C | MWM 2–6 days | Impaired: Surgery Improved: Surgery + Compound C | [84] |
Mice C57BL/6 | Age 18 mos | Isoflurane | Splenectomy | Glycyrrhizin | MWM 14–18 days | Impaired: Surgery Not impaired: Surgery + Glycyrrhizin | [85] |
Rats Sprague-Dawley | Age 18–19 mos | Chloral hydrate, i.p. | Laparotomy | Minocycline | MWM 1, 3, 7 days | Impaired: Surgery; Surgery + Minocycline | [86] |
Mice C57BL/6 | Age 13–14 mos | Sevoflurane | Laparotomy | High-fat diet | MWM 2–9 days | Impaired: Surgery; Surgery + high-fat diet; High-fat controls | [87] |
Mice C57BL/6 | Age 16 mos | Isoflurane Pentobarbital sodium ( ICV implants) | Laparotomy ICV cannula implantation | L-798,106 ICV injection | OF FC 6 days | Impaired: Surgery Not impaired: Surgery + L-798,106 | [88] |
Note: Papers above included (1) preexisting vulnerability in the animal model (age, Alzheimer transgenic, metabolic syndrome), (2) wild-type mice at least 12 months of age, (3) type of anesthesia, (4) type of surgery performed, (5) cognitive testing conducted. PO = postoperative; pre-op = preoperative; ADTg = Alzheimer transgenic; LCR = low-capacity runner; HCR = high-capacity runner; OF = open field; PAT = passive avoidance test; MWM = Morris water maze; FC = fear conditioning; NOR = novel object recognition; OF = open field; RAM = radial arm maze, EPM = elevated plus maze; BM = Barnes maze; ICV = intracerebroventricular; SQ = subcutaneous; i.p. = intraperitoneal; i.v. = intravenous.
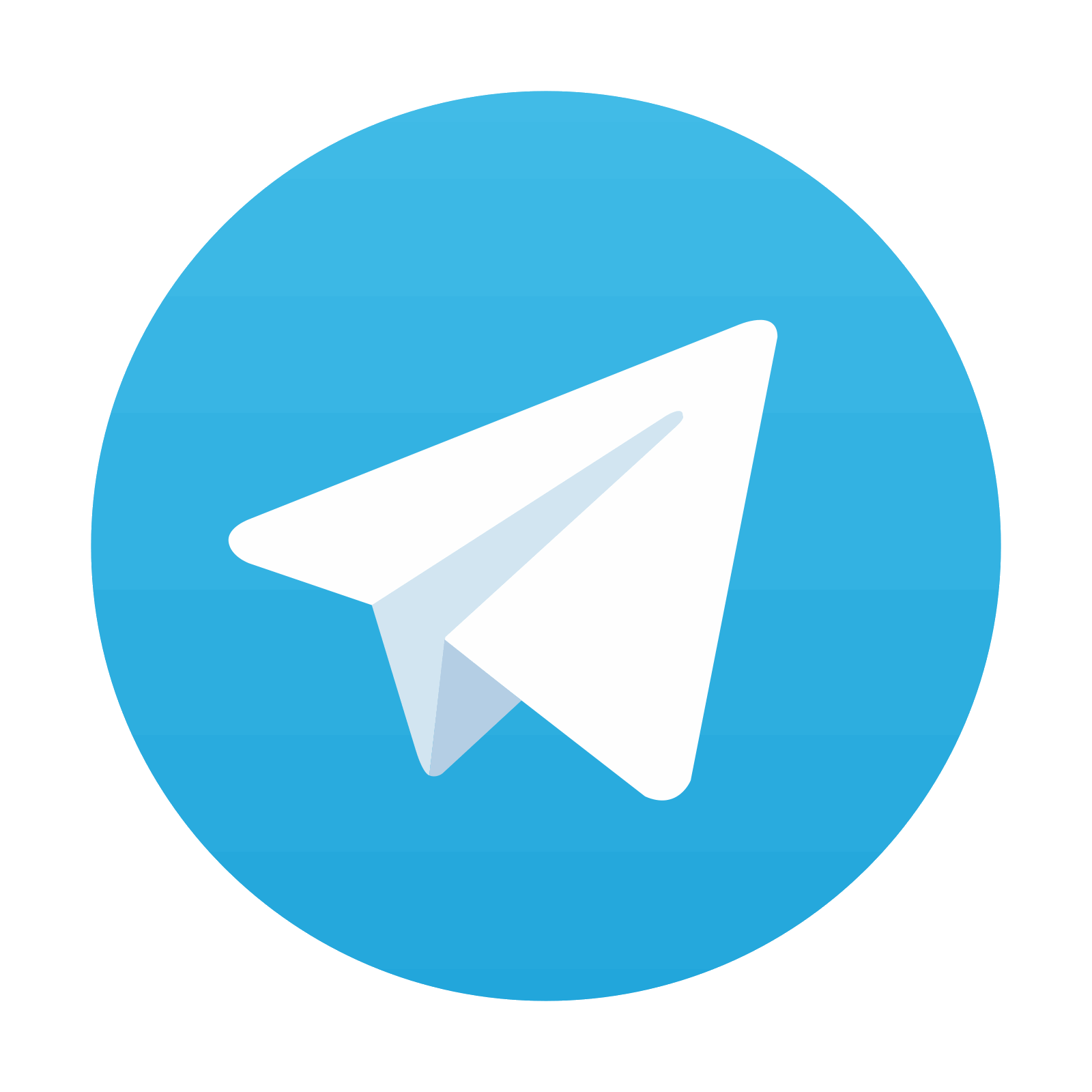
Stay updated, free articles. Join our Telegram channel
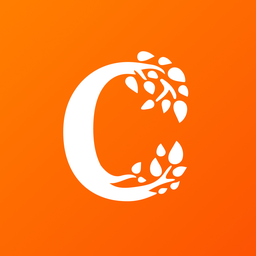
Full access? Get Clinical Tree
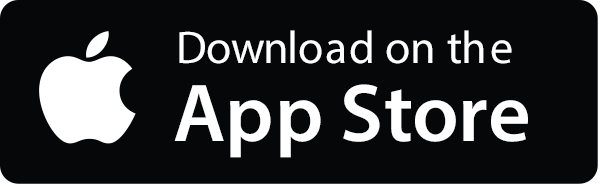
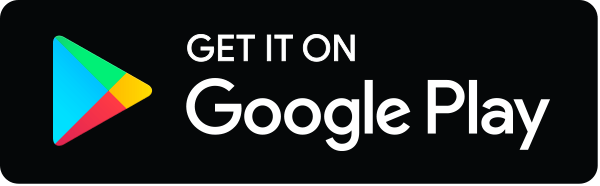
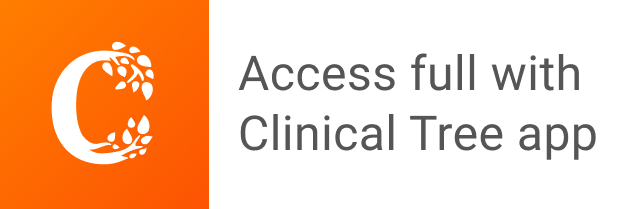