Introduction
The most apparent difference between children and adults is size, but along with anatomical differences, significant variations exist also in pharmacokinetics and pharmacodynamics of the drugs. Rapid growth and development in early years may lead to alteration in uptake, distribution, metabolism, and elimination of drugs. These pharmacokinetic and -dynamic differences in pediatric population depend on age, developmental stage, and genetic factors (see Chapter 1).
Body composition determines the volume of distribution of hydrophilic and lipophilic drugs. Neonates and infants have greater total body and extracellular water, resulting in increased distribution volume for water-soluble drugs. Body fat content starts increasing at birth, peaks at 9 months, and then again starts decreasing. Decreased plasma protein binding may result in increased free drug, resulting in enhanced effect. Glomerular filtration rate and renal tubular secretion reaches the adult level by 8–12 and 6–12 months, respectively. Several anesthetic drugs depend on the liver for clearance. During the first 3 months, activity of hepatic cytochrome enzymes is markedly low and gradually increases to adult levels by 1 year. The hepatic blood flow is also low in neonates.
The minimum alveolar concentration (MAC) of halogenated agents rises during the first month and peaks at 1 year, after which it starts declining. Sevoflurane is an exception as MAC is highest in neonates.
For the ethical constraints, most of the evidence for usage of drugs in children has been extrapolated from results obtained from studies carried out in adults. The anesthetic management of children undergoing neurosurgery requires knowledge of drug actions on different systems (especially on central nervous system) and of its metabolism. In this chapter we primarily discuss the common drugs used in neuroanesthesia practice. The neurotoxicity of these anesthetic drugs is discussed elsewhere in the book (see Chapter 19).
Inhaled Anesthetic Agents
Inhaled anesthetics are the most common agents used for induction and maintenance of anesthesia in children. Presence of anatomical and physiological differences affects wash-in of inhalational agents, therefore it varies with age. Infants have a higher wash-in as compared to older children and adults. Agents that are less blood soluble are affected the least.
Nitrous oxide is highly insoluble in blood, which enables its rapid wash-in and wash-out. It has an anesthetic sparing and analgesic action when combined with other agents. Table 4.1 summarizes the properties and systemic effects of nitrous oxide. An ongoing controversy regarding its use especially in neurosurgical patients arises from the fact that it increases cerebral blood flow (CBF), cerebral metabolic rate (CMRO2), and intracranial pressure (ICP) and impairs cerebral autoregulation. Carbon dioxide (CO2) reactivity is preserved during nitrous oxide anesthesia. The vasodilatory effect can be countered by hyperventilation and administration of intravenous anesthetic agents like propofol and thiopentone. However, in clinical use, much of these effects are variable and depend on the volatile agent being concomitantly used and level of arterial CO2. Rise in ICP makes it a less approved agent for use in conditions with reduced intracranial compliance, though it has not been confirmed by various studies. Use of nitrous oxide has also not shown to increase the incidence of venous air embolism in sitting position, but it must be discontinued as soon as it is detected.1 Nitrous oxide has the ability to diffuse into air containing cavities and expand them. Omission of nitrous oxide does not warrant that pneumocephalus will not develop.2 Some amount of intracranial air may persist for several days even after surgery is completed.3 For this reason, it should be avoided for 4–6 weeks in cases of redo craniotomy, lest it expand the air cavity before dura is opened. There is no literature available on use of nitrous oxide in children having cerebral ischemia. Results extrapolated from animal studies and adult human population show no deleterious effect of its use on neurologic function.4, 5 During intraoperative recording of evoked potentials, it is best to omit nitrous oxide as it can reduce the signal amplitude. Prolonged exposure to nitrous oxide inhibits vitamin B12-dependent enzymes affecting myelin formation, DNA synthesis, homocysteine, and folate metabolism. A short intraoperative exposure is unlikely to result in toxicity. However, it should be avoided in children having methionine synthetase deficiency. On the basis of the current literature, there is still no strong evidence to discontinue nitrous oxide in pediatric neurosurgical cases.
Anesthetic Agent | MAC (2 yrs) | Blood-Gas Partition Coefficient | % Metabolized | MAP | CBF | ICP | CMRO2 | CPP | SSEP | MEP |
---|---|---|---|---|---|---|---|---|---|---|
Nitrous oxide | Unknown | 0.47 | — | 0 | ↑ | ↑ | ↑↓ | ↓ | 0↓ | ↓ |
Halothane | 0.97 | 2.4 | 20 | ↓ | ↑↑ | ↑↑ | ↓ | ↑↑ | ↓ | ↓ |
Isoflurane | 1.6 | 1.4 | 0.2 | ↓ | ↑ | ↑ | ↓ | ↑ | ↓ | ↓ |
Sevoflurane | 2.6 | 0.66 | 5 | ↓ | ↑ | 0–↑ | ↓ | ↑ | ↓ | ↓ |
Desflurane | 8.7 | 0.42 | 0.02 | ↓ | ↑ | ↑ | ↓ | ↑ | ↓ | ↓ |
Xenon | Unknown | 0.115 | 0 | 0 | ↑↓ | ↑ | ↓ | ↑ | Unknown | Unknown |
MAC = minimum alveolar concentration; MAP = mean arterial pressure; CBF = cerebral blood flow; ICP = intracranial pressure; CMRO2 = cerebral metabolic rate of oxygen; CPP = cerebral perfusion pressure; SSEP = somatosensory evoked potential; MEP = motor evoked potential; ↓ = decreases; ↑ = increases; 0 = no effect.
Halogenated Anesthetic Agents
The mechanisms of action of these agents include antagonism of N-methyl-D-aspartic acid (NMDA) receptor, activation of gamma-aminobutyric acid (GABA) and glycine receptors, inhibition of ionic channels, and alteration of cellular proteins. All volatile inhalational agents decrease systemic blood pressure and increase heart rate in a dose-dependent manner (except halothane, which causes an increase in heart rate). All of these depress ventilatory drive and decrease tidal volume. All produce bronchodilatation, but evidence is not strong enough for desflurane.6 They may all trigger malignant hyperthermia in susceptible children. Preconditioning with isoflurane, sevoflurane, and desflurane provides neurologic protection. However, all of them have also been implicated in neurotoxicity though to a varying extent.
Isoflurane is the most common volatile anesthetic for the maintenance of general anesthesia. However, it is not used for induction of anesthesia as it has a pungent odor that may irritate the airway and may even cause laryngospasm. All halogenated volatile anesthetic agents increase CBF and decrease CMRO2. At 1 MAC, CO2 reactivity and cerebral autoregulation is preserved. It does not affect CSF production but facilitates CSF reabsorption. At a low concentration, it increases the EEG frequency and decreases the voltage. At higher concentrations, it decreases frequency and increases amplitude. It can even produce burst suppression and isoelectric pattern on electroencephalography (EEG). Isoflurane is the most potent suppressant of cortical somatosensory evoked potentials (SSEPs) and should be used in concentration <0.5 MAC for successful recording. Motor evoked potentials (MEPs) are diminished even at 0.2–0.5 MAC isoflurane. Many experimental and clinical models of cerebral ischemia suggest that isoflurane exerts a neuroprotective effect.7, 8
Sevoflurane has been the gold standard agent for inhaled inductions because of its pleasant and nonirritating scent and low blood solubility, enabling its rapid uptake and wash-off. The recovery profile is better with sevoflurane as compared to isoflurane.9 Rapid wash-off of sevoflurane at time of awakening may cause emergence delirium, which is comparable to that of desflurane.10 It causes dose-dependent cerebral vasodilation resulting in raised CBF. It decreases CMRO2 and preserves cerebrovascular reactivity to CO2. It maintains cerebral autoregulation up to 1.5 MAC. Epileptiform EEG pattern has been seen at high concentration of sevoflurane.11 The age-related brain developmental process like synaptogenesis and myelination affects the electroencephalogram (EEG) dynamics in response to sevoflurane especially in children less than 1 year.12 The degradation of sevoflurane to produce inorganic fluoride and compound A rarely results in problems in clinical practice. Sevoflurane decreases the amplitude and increases latency of SSEPs, albeit at a higher concentration of 1–1.5 MAC. Sevoflurane has an action similar to isoflurane on MEPs. Under specific conditions it produces antiapoptotic effects, increased mitochondrial phosphorylation, activation of K (TREK-1) channel, and inhibition of caspase-3, thus imparting neuroprotection.13–15 Sevoflurane can be associated with a higher incidence of emergence delirium when compared to other halogenated anesthetics.16
Desflurane has the most rapid wash-in and wash-off among the available inhalational anesthetic agents owing to its lowest blood:gas partition coefficient. It is not used for induction of anesthesia as it may incite laryngospasm and breath holding because of its pungent odor. It boils at room temperature (23.5°C), so requires a special heated vaporizer for its delivery. Similar to other volatile agents, it causes cerebral vasodilatation, impairs autoregulation at 1.0 MAC, increases ICP, and preserves CO2 reactivity. Isoflurane, sevoflurane, and desflurane 0.5–1.0 MAC in nitrous oxide when administered to children all increase ICP and decrease minimum alveolar concentration (MAP) and cerebral perfusion pressure (CPP) in a dose-dependent manner.17 The degree of increase in ICP with various agents is in the following order: desflurane >isoflurane > sevoflurane.18 It suppresses EEG activity and can induce burst suppression at 1.24 MAC.19 At 1.5 MAC desflurane, SSEP and MEP are suppressed. The amount of carbon monoxide production in the presence of desiccated soda lime or baralyme is greatest with desflurane and least with sevoflurane. Neuroprotection and cardioprotection with desflurane has been observed in several studies.20, 21 While few studies report more neuroapoptosis with desflurane than sevoflurane or isoflurane in neonatal animal studies, others are equivocal.22, 23
Intravenous Anesthetic Agents
Most intravenous anesthetic agents exert their effect by binding to different subunits of gamma-aminobutyric acid type A (GABAA) receptors and potentiating their inhibitory action. GABAA receptors form chloride channels, and when activated, they lead to postsynaptic hyperpolarization and inhibition of neuronal activity. The cerebrovascular effects of various agents are depicted in Table 4.2.
Anesthetic Agent | MAP | CBF | ICP | CMRO2 | CPP | SSEP | MEP |
---|---|---|---|---|---|---|---|
Sodium thiopentone | ↓↓ | ↓↓↓ | ↓↓↓ | ↓↓↓ | ↑↑↑ | ↓A↑L | ↓↓ |
Propofol | ↓↓↓ | ↓↓↓ | ↓↓ | ↓↓↓ | ↑↑ | ↓A | ↓ |
Etomidate | 0–↓ | ↓↓ | ↓↓ | ↓↓↓ | ↑↑ | ↑A | ↑ |
Ketamine | ↑ | ↑↑ | ↑↑ | ↑ | ↓ | ↑A | 0 |
Benzodiazepines | 0–↓ | ↓↓ | 0 | ↓↓ | ↑ | 0 | ↓↓↓ |
Opioids | 0–↓ | ↓ | 0–↓ | ↓ | ↓↑ | ↓A↑L | 0 |
MAP = mean arterial pressure; CBF = cerebral blood flow; ICP = intracranial pressure; CMRO2 = cerebral metabolic rate of oxygen; CPP = cerebral perfusion pressure; SSEP = somatosensory evoked potential; MEP = motor evoked potential; ↓ = decreases; ↑ = increases; 0 = no effect; A = amplitude; L = latency.
Sodium thiopenthal is an ultrashort-acting barbiturate used as an induction agent. It is highly lipid soluble and protein bound (80%). It is a direct myocardial depressant and decreases MAP, resulting in baroreceptor activation and an increase in heart rate. The degree of blunting of airway reflexes is less than propofol, predisposing to laryngospasm and bronchospasm. It has a favorable profile by decreasing CBF, cerebral blood volume, CMRO2, and ICP. It does not impair cerebral autoregulation and CO2 reactivity. It decreases the amplitude and latency of cortical SSEPs and inhibits MEP. It has been found to have a neuroprotective effect in the treatment of focal cerebral ischemia. The induction dose of sodium thiopental in neonates (ED50=3.4 mg/kg) is less than in infants (ED50=6.3 mg/kg).24 Small children have more vessel-rich tissue, which hastens uptake and achieves a rapid effect and prompt redistribution and elimination. Early awakening after a single intravenous bolus occurs by redistribution from brain to other tissues. It may also be administered as an infusion in children having refractory epilepsy. The dose is 3–5 mg/kg bolus, followed by an infusion of 3–5 mg/kg/hr. Hypotension is an important side effect and requires administration of vasopressors. Elimination from adipose tissue may be prolonged after administration of continuous infusion. Porphyria is an absolute contraindication to barbiturate administration.
Propofol is available as 1% aqueous emulsion containing soya bean oil, egg lecithin, and glycerol. The addition of disodium edetate helps in controlling bacterial growth. It is rapidly redistributed and metabolized by liver glucuronidation and other extrahepatic mechanisms. It decreases cardiac contractility, systemic vascular resistance (SVR), and preload, resulting in hypotension and reactionary increase in heart rate. It depresses the respiration and obtunds upper airway reflexes. It decreases CBF, CMRO2, and ICP. Cerebral autoregulation and CO2 reactivity are preserved. As a part of a total intravenous anesthesia technique, it is the preferred agent for somatosensory and MEP monitoring.25 Propofol is helpful in controlling seizures and may be administered as an infusion. It has an additional advantage of having an antiemetic effect. ED50 of propofol for older children is 2.4 mg/kg and for infants is 3 mg/kg. However, these doses can lead to hypotension for as long as 30 minutes in unstimulated neonates and infants. Propofol infusion more than 10mg/kg/hr lasting for more than 48 hours if administered in critically ill patients may result in propofol infusion syndrome,characterized by metabolic acidosis, rhabdomyolysis, and renal and heart failure. For this reason, and associated hypotension, propofol is not used for intensive care unit (ICU) sedation in pediatric head injury patients. Pain on injection is a common side effect, and thus it is often mixed with lidocaine to reduce pain intensity.
Etomidate is a short-acting nonbarbiturate agent used primarily for induction of anesthesia. When used for this purpose it causes negligible hemodynamic perturbations but is commonly associated with myoclonic movements. It is also associated with pain at the injection site, which can be minimized by use of a formulation containing medium- and long-chain triglycerides. The cerebrovascular action comprises reduction of CBF, CMRO2, and ICP. Absence of negative ionotropic effect maintains the blood pressure, which is advantageous in setting of intracranial hypertension.26 It increases the amplitude of cortical SSEP and MEP during evoked potential monitoring. Etomidate has proconvulsant action and can produce epileptiform discharges. A major side effect is adrenal suppression, which may occur after continuous infusion or even a single induction dose. It blocks 11β-hydroxylase, thus inhibiting steroid synthesis. The dose used is 0.2–0.3 mg/kg. A pyrrole analog of etomidate known as carboetomidate does not affect steroid synthesis as well as maintains hemodynamic stability.27
Ketamine is an effective sedative and analgesic that produces dissociative anesthesia. It is a noncompetitive NMDA receptor antagonist. It preserves the upper airway reflexes and maintains respiration and blood pressure. The dose is 2 mg/kg, and requirement increases with decreasing age. Below the age of 3 months, its metabolism and clearance are reduced and half-life is prolonged. It can be administered through oral, intravenous, and intramuscular routes. It is a cerebral vasodilator and increases intraocular pressure (IOP), CBF, CMRO2, and ICP. For these reasons it is generally not preferred in neurosurgical patients at risk of intracranial hypertension. Ketamine increases the amplitude of cortical SSEP and enables monitoring of myogenic MEP. It produces electroencephalographic seizure activity at low doses by blocking the inhibitory interneurons. At higher doses, it also blocks the excitatory neurons, thus acting as an anticonvulsant. It has been used as an effective antiepileptic in pediatric refractory status epilepticus patients without causing any complications.28 However, significant controversy exists regarding its effect on ICP and neurotoxicity. Ketamine has even been shown to possess ICP-lowering action when administered to patients of head trauma being ventilated and sedated with propofol.29 The effect of ketamine on ICP in severe head injury patients was assessed in 101 adult and 55 pediatric patients with severe head injury. ICP did not increase during ketamine administration when patients were sedated and ventilated.30 On the basis of the available evidence, ketamine administration increases global CBF and rCBF in humans, but the level of evidence is 2b (grade C). Thus, it should not be routinely prohibited in all neurologically ill patients.31 Ketamine may provide neuroprotection by virtue of NMDA receptor antagonism, antiapoptotic effect, and interference with the inflammatory reaction to injury. In several animal models, it has been shown to induce neuroapoptosis, but this has not been confirmed in humans who clinically receive much lower dose and exposure time. Hallucinations, euphoria, and dysphoria are common excitatory reactions seen after ketamine administration and may be lessened by coadministration of midazolam.
Benzodiazepines are GABA agonists that produce sedation and amnesia. Midazolam is a short-acting benzodiazepine that is often used as a premedicant, sedative, and inducing and antiepileptic agent. It causes anxiolysis, sedation, and anterograde amnesia, which makes it an ideal premedicant. It causes respiratory depression in a dose-dependent manner. It decreases SVR and blood pressure and increases heart rate. It decreases CBF and CMRO2 but has a plateau effect beyond which it cannot further decrease CMRO2 and produce isoelectric EEG. CO2 reactivity is maintained and has no effect on ICP. It can be administered through various routes like oral, sublingual, intranasal, intravenous, intramuscular, and rectal. Midazolam has minimal effect on cortical SSEP but suppresses MEP even at small doses.25 It is an effective anticonvulsant and can be used as an infusion for control of status epilepticus in children.32 Dose for intravenous bolus and oral use is 0.05–0.1 mg/kg and 0.3–0.75 mg/kg. Low incidence of paradoxical reactions may be observed in children, which may require administration of other agents like propofol, ketamine, and benzodiazepine antagonist flumazenil (0.3–0.5 mg). However, flumazenil should be avoided in children having a history of seizures or elevated ICP because rapid reversal of midazolam effect may lead to a rebounding increase in CBF and ICP.
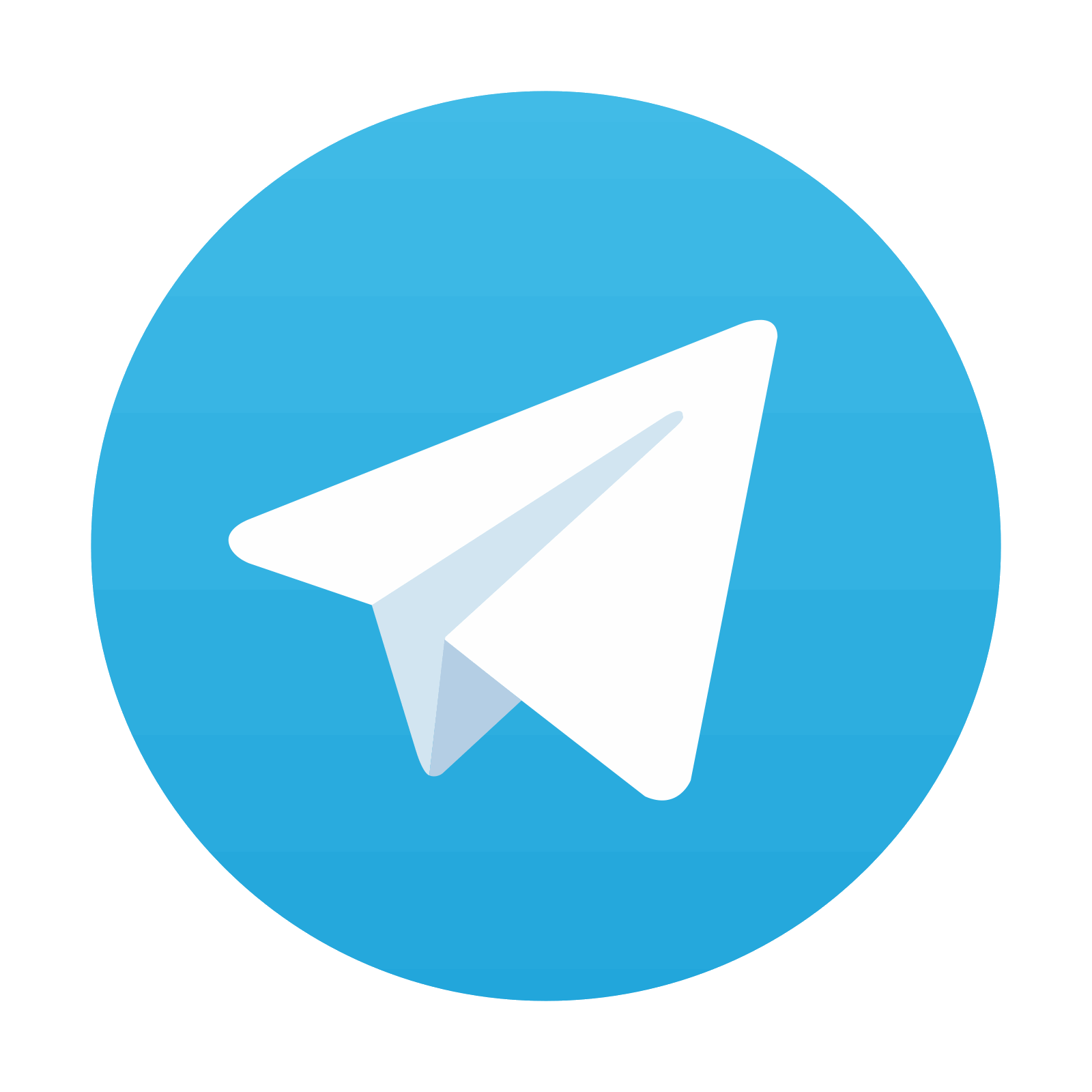
Stay updated, free articles. Join our Telegram channel
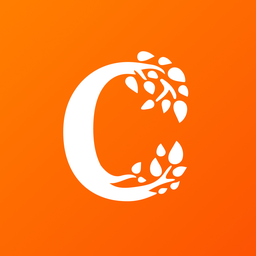
Full access? Get Clinical Tree
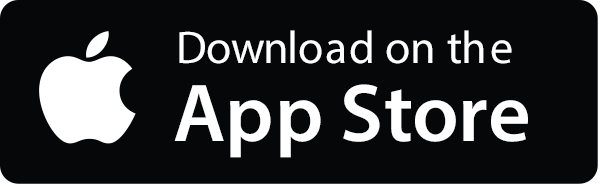
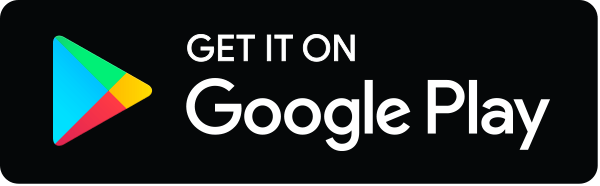
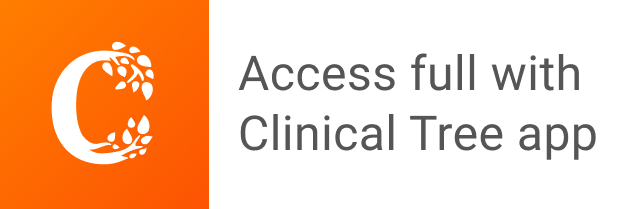