Introduction
The pediatric brain utilizes a delicate homeostatic balance to ensure proper regional blood flow for nutrient delivery and waste removal. When homeostatic mechanisms are dysfunctional, normal neuronal activity and development are compromised, predisposing the maturing brain to morbidity. In the perioperative environment, pediatric cerebral injury may include focal (regional stroke) or global (hypoxic-ischemic encephalopathy; HIE) damage, resulting from surgical factors and alterations to control mechanisms induced by disease/patient or iatrogenic considerations. Over the course of the past 60 years, with the development of preclinical models of brain injury, our understanding of the mechanisms underlying this phenomenon has increased remarkably, as has our understanding of therapeutic interventions conferring a neuroprotective benefit. Neuroprotection refers to the preservation, recovery, or regeneration of neuronal tissue, and diverse perioperative interventions have been investigated for their neuroprotective benefit.
Mechanisms of Brain Injury in the Perioperative Setting
In the pediatric population, the primary initiators of neuronal injury in the perioperative setting are ischemia/hypoxia and inflammation, creating local excitotoxicity and the generation of reactive oxygen species (ROS) that potentially extend damage outside the initial at-risk area.1, 2 Global exposure to hypoxia, a particular concern for infants with less developed cerebral autoregulation, can lead to the development of HIE, epilepsy, cerebral palsy, and mild to severe developmental delay.3
During ischemia/hypoxia, the cell has a relative deficiency of oxygen, reducing high-efficiency aerobic metabolism (mitochondrial) in favor of lower efficiency anaerobic metabolism, and disrupting the cell’s ability to maintain normal homeostatic balance. Neurons have a particularly high energetic demand to maintain membrane potential, and the failure of important ionic pumps in the cell membrane that normally consume significant ATP can occur during hypoxia (i.e., Na/K ATPase), leading to cell depolarization and excess release of glutamate,4 the primary excitatory neurotransmitter. Increased glutamate can trigger an excitotoxic cascade, via activation of N-methyl-D-aspartate (NMDA) and alpha-amino-3-hydroxyl-5-methyl-4-isoxazole-propionate (AMPA) receptor,5 characterized by calcium influx into the cell, further mitochondrial dysfunction, increased intracellular nitric oxide, and the production of reactive oxygen and nitrogen species.2, 4, 5 Irreversible cell damage from excitotoxicity, progressing over a period of minutes to days, can trigger apoptotic pathways, resulting in cell death both in and around the original area that experienced the oxygen/nutrient deficit.5
The developing brain is particularly sensitive to excitotoxic damage.4 In preterm infants, between 23 and 32 weeks of gestational age, the release of excitotoxic neurotransmitters can lead to oligodendrocyte progenitor and neuronal cell death1, 2, 4 and white matter injury.1, 2 This susceptibility to excitotoxicity is relevant for anesthesia in premature infants, as the gamma-aminobutyric acid type A (GABA-A) channel is excitotoxic through parts of development, and inhalational anesthetics may then exacerbate neuronal damage. Peroxisomes are used to help clear ROS in the cell, and are needed for the maturation of oligodendrocyte progenitor cells.2 Interestingly, inflammation in the developing brain can reduce peroxisomal proliferation and prevent oligodendrocyte maturation, as a potential mechanism for white matter damage and adverse changes to developing nerve myelination.2 Through the described mechanisms, ischemia/hypoxia, excitatory neurotransmitters, and inflammation form a damaging triad, each being caused by and exacerbating the other to induce neuronal dysfunction and death. Strategies to protect the pediatric brain during the perioperative period then focus on the prevention of hypoxia, and the mitigation of excitotoxicity, oxidative damage, and inflammation.
Physiologic Neuroprotective Interventions
Under anesthesia, mechanisms of cerebral autoregulation may be impaired, and oxygen/nutrient supply and demand must be matched to prevent ischemic or hypoxic injury. To this end, physiologic interventions, such as hyperventilation, osmodiuresis, glucose management, and hypothermia, are frequently used interventions in neuroanesthesia management.
As intracranial pressure (ICP) accumulates, cerebral perfusion may fall due to the fixed volume of the calvarium (with the Monro-Kellie doctrine, cerebral blood volume and perfusion are compromised at the expense of the enlarging brain).6 Increasing ICP also compromises the ability of the local vasculature to regulate blood flow. As such, maintenance of normal ICP is a cornerstone of neuroanesthetic management to ensure both regional (in particular watershed) and global cerebral nutrient supply-demand matching. Reducing ICP may be accomplished by a number of mechanisms, including osmodiuresis, patient positioning (reverse Trendelenburg), and hyperventilation/hypocapnea.
Hyperventilation with hypocapnea induces a transient reduction in cerebral blood volume and blood flow in an attempt to emergently reduce ICP and (somewhat paradoxically) improves neuronal nutrient supply-demand by partially restoring autoregulation. Deliberate hyperventilation to an arterial carbon dioxide pressure of 25 to 30 mm Hg is a standard of care in the management of increased ICP.7 Chapter 2 provides a comprehensive discussion of the effect of hypocapnea on cerebral blood flow.
Osmodiuretics are employed in neuroanesthesia to reduce ICP, provide brain relaxation, improve surgical conditions, and prevent neurologic deterioration. The effectiveness of osmodiuretics is linked to their “reflection coefficient,” which defines the relative ability of the agent to cross the blood-brain barrier (where a coefficient of 0 indicates free flow across the blood-brain barrier, and a coefficient of 1 indicates complete impermeability). Mannitol and hypertonic saline are two common osmotic diuretics used in neuroanesthesia. The reflection coefficient of mannitol is 0.9 and that of hypertonic saline is 1.0, indicating that hypertonic saline may be a superior osmodiuretic to mannitol, producing less rebound cerebral edema with agent removal.8 Recently, it has been demonstrated that hypertonic saline preserves cerebral microcirculation in an animal model,9 and is more effective than mannitol in reducing ICP during neurosurgical procedures.8 All osmodiuretic agents may be less effective in damaged areas of the brain since the reflection coefficient in that area may be altered, adversely allowing for the agent to cross the vasculature.
Patient positioning can also impact ICP. The use of 10 degree reverse Trendelenburg position has been shown to reduce ICP and, in pediatric patients undergoing craniotomy, the supine position has been demonstrated to reduce ICP compared to the prone position.10
To prevent neuronal damage not necessarily associated with an increased ICP (like neonatal hypoxic brain injury), there is strong evidence for the neuroprotective benefit of hypothermia.11 Multiple randomized trials have demonstrated the effectiveness of therapeutic hypothermia initiated within 6 hours of neurological injury in reducing neurodevelopmental disability at 18 months of age in the setting of moderate to severe HIE,11 a benefit that appears to be preserved into middle childhood.12 The mechanisms underlying this neuroprotection are thought to include a reduction of cerebral metabolic rate (better balancing of nutrient supply-demand) and a reduction of excitotoxic free radicals.12 The beneficial effect of hypothermia for pediatrics contrasts with adult data, where hypothermia does not consistently show a beneficial effect for neuroprotection during anesthesia or outside the operating room.13 This difference may be from the increased neuronal plasticity in the young, or may be related to the underlying physiology where (micro)vascular disease would be much more prominent in the adult population and flow would be quicker to cease and take longer to return.
Hyperthermia, however, has been associated with brain injury.6 Hyperthermia may increase metabolic demand, depleting energy stores and increasing cerebral blood flow, which may exacerbate reperfusion injury.6 Elevated temperature has been associated with excitotoxic glutamate release independent of neuronal cell death, the generation of free radicals, and the elevation of neuronal inflammatory markers. Preclinical studies have demonstrated that hyperthermia exacerbates ischemic brain injury, and human data in pediatrics and adults support this association.6 The avoidance of hyperthermia is therefore imperative in neurosurgical anesthesia.
Hypoglycemia and hyperglycemia are both associated with poor neurologic outcomes in ischemic cerebral injury.14 Elevated glucose levels in acute ischemic stroke are predictive of increased infarction size, more significant reperfusion injury, the production of proinflammatory mediators and ROS, and increased mortality.14 Glucose is the most important energy substrate for the brain, and the majority of glucose uptake, under basal conditions, is used for maintenance of normal ionic balance. Seizures and hypoglycemic coma can ensue as blood glucose drops to 2.8 to 3.0 mmol/L, and neuronal death occurs in animal models when the electroencephalography (EEG) becomes isoelectric. Hypoglycemia increases excitatory neurotransmitters in the extracellular space, and generates ROS. As an example of the importance of glucose homeostasis in neuronal survival and function, it was observed that after ischemic stroke in adults, both high and low glucose level on admission correlated with poorer neurocognitive outcomes at 24 hours, with the optimal glucose at 5 mmol/L.15 A prospective study in pediatric cardiac surgery, however, found an association between intraoperative hypoglycemia but not hyperglycemia with electroencephalographic seizures and slower electroencephalogram recovery, although long-term neurocognitive outcomes were unaffected in both groups.16 Regardless, maintenance of reasonable normoglycemia during neuroanesthesia is advocated.
Ischemic conditioning refers to the application of a subtoxic ischemic challenge to a tissue, serving to limit cellular damage and death from a more lethal ischemic event through alterations to cellular physiology.17 Ischemic conditioning may be performed prior to, during, or after an insult, referred to as ischemic pre-, per-, and postconditioning, respectively. While all three work via similar (but not exact) mechanisms, there are differences in the protection benefit they provide for a certain type of insult or stress. Remote ischemic preconditioning has demonstrated effectiveness in animal models,17 conferring benefit through overlapping biomolecular pathways, including antioxidative and antiapoptotic effects, subcellular organelle-based ischemic tolerance (especially mitochondrial), downregulation of inflammatory mediators, and glutamate and NMDA receptor-mediated neuroprotection. Preconditioning initiated after the induction of anesthesia but prior to surgical insult can be effective in reducing postoperative cognitive impairment.18 An intervention with good feasibility, remote ischemic per-conditioning has been shown to reduce infarction when used as an adjunctive treatment in adult ischemic stroke.19 Although more research is needed for routine practical implementation, remote ischemic conditioning shows promise as a neuroprotective strategy.
Pharmacologic Neuroprotective Interventions
Anesthetic Neuroprotection
The ability of anesthetic agents to protect the brain against neuronal injury was first identified in the 1960s, when it was demonstrated that general anesthesia reduced electroencephalogram seizure activity in the setting of carotid artery occlusion.20 At the time, it was hypothesized that a decrease in cerebral metabolic rate with the use of anesthesia played a significant role in the observed neuroprotection. Since then, a considerable body of research has further characterized neuroprotection from volatile and intravenous anesthetic agents, and the mechanisms underlying their effect. Recently, however, concerns over anesthetic neurotoxicity have also emerged, focusing ongoing research toward the identification of the determinants of neuroprotection and neurotoxicity and how the causative pathways overlap (see Chapter 19).
Several biomolecular mechanisms have been implicated in anesthetic conditioning against ischemic cerebral injury.21 Anesthetic agents stimulate inhibitory GABA receptors, preventing membrane depolarization and energy expenditure during ischemia, thereby reducing excitotoxicity.21 Isoflurane reduces the release of glutamate during cerebral ischemia, and the drug has been linked to a reduced response to both NMDA and AMPA in neurons. Activation of mitochondrial potassium ATP (mitoKATP) channels has also been shown to be enhanced by volatile anesthetics, leading to mitochondrial membrane hyperpolarization and inhibition of metabolic expenditure.21 Exposure to volatile and intravenous anesthetics has been associated with an upregulation of antiapoptotic factors and a reduction of apoptotic cell death, via mitochondrial and calcium-dependent mechanisms. Other implicated pathways for anesthesia neuroprotection include an upregulation of antioxidant enzymes, a reduction of cerebral oxidative stress, diminished central nervous system catecholamines, and an increase in cerebral blood flow.21–23
Neuroprotection by Volatile Anesthetic Agents
The neuronal protection conferred by volatile anesthetic agents to hypoxia/ischemia has been well established in preclinical studies. In animal models of cerebral ischemia and excitotoxicity, both short- and long-term neuroprotection have been demonstrated with global and focal insults. Although robust evidence for improved short-term neurocognitive outcomes with the use of inhalational anesthetics exists, consensus on the long-term neuroprotective effects of volatile agents has yet to be reached.21 Inhalational agents can confer neuroprotection when administered prior to, during, or shortly after a neurotoxic insult (similar to ischemic conditioning, referred to as pre-, per-, and postconditioning). Current evidence does not support the use of significantly delayed (>1 hour) anesthetic postconditioning. Whether volatile anesthetics provide neurocognitive protection in the clinical setting is the subject of ongoing research.21 In the pediatric population, where concerns of volatile anesthetic agent neurotoxicity in the developing brain exist (see Chapter 19), the elucidation of factors mediating both neurotoxicity and neuroprotection of anesthetic agents is of prime importance.
The neuroprotective properties of isoflurane have been well documented in the literature.24–26 Isoflurane has been demonstrated to provide dose-dependent neuroprotection in in vitro and in vivo models of cerebral ischemia when used prior to, during, or after the ischemic insult.23, 25 Exposure to a GABA-A receptor antagonist markedly attenuates the neuroprotection conferred by isoflurane, suggesting a role for the receptor in isoflurane’s beneficial effect (not off-target). Long-term neurocognitive benefit from isoflurane conditioning, however, remains uncertain. Several groups have demonstrated the lack of long-term neurocognitive or histologic benefit from isoflurane as early as 3 weeks post-ischemic injury.21, 24
As with isoflurane, sevoflurane preconditioning prior to focal or global ischemia demonstrates a neuroprotective effect in a dose-dependent manner.23 Immediate postconditioning with sevoflurane, resulting in reductions in infarct size and apoptotic cell death and improvement in neurocognitive function, has been demonstrated.27 Long-term neurocognitive benefit, persisting up to 4 weeks after ischemic injury, was seen with sevoflurane alone, or in combination with xenon.27 Neuroprotection with the volatile anesthetic desflurane has received less attention, but available evidence demonstrates similar neuroprotection to other inhalational agents. When administered prior to an ischemic insult in animal models, desflurane demonstrated greater neuroprotective benefit than halothane, but comparable to isoflurane.26, 28
Nitrous oxide is an inhalational anesthetic agent with anti-NMDA properties.29 In vivo models have described both the neuroprotective and neurotoxic effects of nitrous oxide, similar to the stroke literature, where NMDA antagonists have been trialed for decades for recovery after an ischemic insult, with mixed results. Nitrous oxide demonstrated dose-dependent neuroprotection from the excitotoxic effects of subcutaneous racemic NMDA injection in rats, and exposure of neonatal rats to 70% nitrous oxide for 6 hours did not result in apoptotic neurodegeneration.29, 30 However, the dose-dependent neurotoxic properties of nitric oxide have also been demonstrated. In one in vitro model, this effect was attenuated by GABAergic drugs such as scopolamine or thiopental, suggesting a GABA-mediated mechanism.29 Overall, however, the body of literature supporting a role for nitrous oxide in cerebral neuroprotection is less robust compared to that for other inhaled anesthetics.
Xenon is a noble gas with general anesthetic properties. As an anesthetic, it exerts its effect primarily via NMDA receptor antagonism, although AMPA and kainite receptors have also been implicated in xenon’s mechanism of action.31 The neuroprotective properties of xenon have been demonstrated in in vitro and in vivo models.23, 31, 32 Xenon reduced neuronal death in the cortex and striatum via an NMDA-dependent mechanism in an in vivo model of ischemic brain injury.32 When administered 1 hour post-global ischemic injury in a rodent model, xenon attenuated damage to neurons.33 In models of HIE secondary to perinatal asphyxia, xenon has been used in combination with hypothermia to provide neuroprotection in in vitro and in vivo animal models.34 A feasibility study investigating the safety of 50% xenon in conjunction with mild hypothermia in infants with neonatal encephalopathy using a closed-circuit delivery system found no adverse respiratory or cardiac effects with xenon treatment.35 Evidence suggests that xenon is an anesthetic agent that may provide significant neuroprotection, warranting further investigations to improve its practical utility.
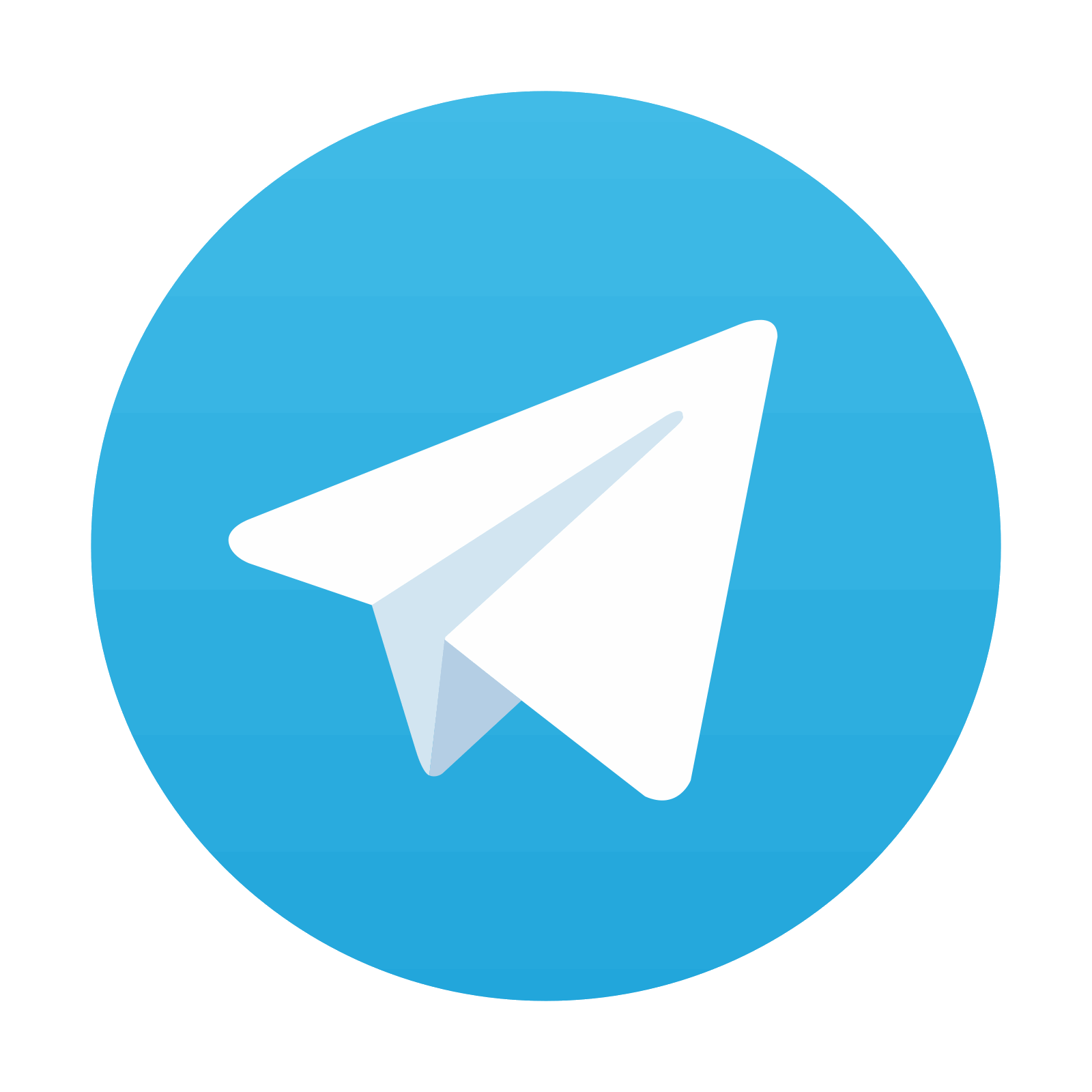
Stay updated, free articles. Join our Telegram channel
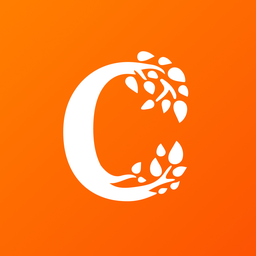
Full access? Get Clinical Tree
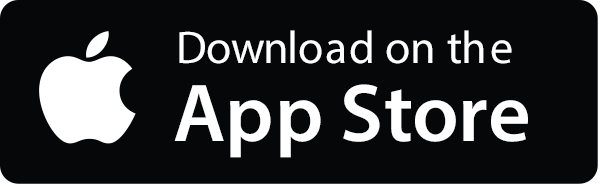
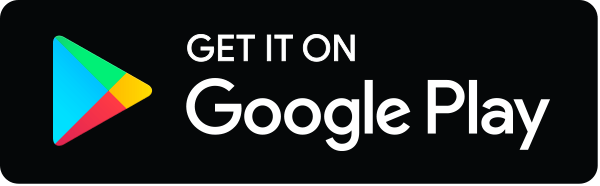
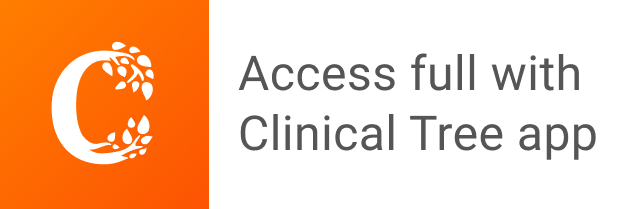