Abstract
The nature and origin of hypoxia is multifaceted. The prevention and treatment include optimisation of pre- and peroxygenation and the wise choice and use of neuromuscular blocking agents. Upper airway reflexes are important to anaesthetists as a clear airway allows safe ventilation of the lungs and oxygenation of the patient. Anaesthetic agents produce changes in the sensitivity of upper airway reflexes, with propofol being associated with depression of upper airway reflexes. This means that airway manipulation, and insertion of airways, including laryngeal mask airways, are more easily tolerated following induction of anaesthesia with propofol compared with other induction agents. Ageing leads to a gradual reduction in the sensitivity of upper airway reflexes. Cigarette smoking increases the sensitivity of upper airway reflexes, a change which persists for up to 2 weeks following cessation of smoking.
Hypoxia
Humans are adapted to tolerate oxygen pressures which range from what might be called ‘sea-level normoxia’, down to the modest hypoxia of high-altitude living. Humans are not adapted to hyperoxic conditions and these are increasingly recognised as harmful. Why then should the airway specialist be concerned about hypoxia, and seek to counter it with hyperoxic exposure?
Classification of Hypoxia
‘Cellular respiration’ occurs at the level of the mitochondria, when electrons are passed from an electron donor (reduced nicotinamide adenine dinucleotide (NADH)) via the mitochondrial respiratory cytochromes to ‘reduce’ molecular oxygen (O2). The energy from this redox reaction is used to phosphorylate adenosine diphosphate (ADP), thereby generating the universal energy source, adenosine triphosphate (ATP), which powers all active biological processes. If molecular oxygen cannot be reduced in this way, this bit of biochemistry fails and cellular hypoxia occurs. Based on Barcroft’s original classification, four separate causes of cellular hypoxia can be considered. Three of these four factors affect oxygen delivery to the tissues (ḊO2), which is described mathematically by the equation in Box 2.1. Derangements of each of the terms on the right-hand side of this equation will reduce oxygen delivery to tissues.
The fourth cause of cellular hypoxia in our classification is histotoxic hypoxia. An example of this is cyanide or carbon monoxide poisoning. In histotoxic hypoxia, there is not (or there need not be) any deficit in oxygen delivery. Cellular and mitochondrial partial pressure of oxygen (PO2) may be more than adequate, but the deficit lies in the reduction of molecular oxygen due to a failure of electron transfer. In order to fully understand the classification of hypoxia, it is useful to consider the example of carbon monoxide poisoning.
What Is the Mechanism of Death in Severe Carbon Monoxide Poisoning?
Let us consider each of the factors of Barcroft’s classification.
Hypoxaemic hypoxia is not likely to be the cause. Assuming no lung damage has occurred, this patient’s arterial oxygen (PaO2) is likely to be normal if breathing air, or elevated if breathing supplemental oxygen. PaO2 is determined by the gas-exchanging properties of the lung and is unaffected by haemoglobin concentration or by the nature of the haemoglobin species present.
Anaemic hypoxia. The presence of carboxyhaemoglobin, which has no oxygen-carrying capacity, will certainly reduce the amount of normal oxygen-carrying haemoglobin. But normal oxyhaemoglobin will still be in the majority, and the ḊO2 will be more than adequate. Counter to popular understanding perhaps, the presence of carboxyhaemoglobin is not the problem here.
Stagnant hypoxia is unlikely to be a cause, since the cardiac output is likely to be elevated as a compensatory mechanism.
So, what is the cause of death? The underlying mechanism of cellular death in this case is histotoxic hypoxia. Just as carbon monoxide has a high affinity for the haem group in haemoglobin, it also has a high affinity for the iron-containing haem flavoproteins in mitochondrial respiratory cytochromes. Once bound, electron transfer is interrupted and tissue oxygen, albeit in abundant supply, cannot be reduced and bioenergetic failure supervenes. In carbon monoxide poisoning, the presence of carboxyhaemoglobin merely serves as a marker of carbon monoxide exposure. It is not usually part of the mechanism of death.
Differential Effects of Deficits in Oxygen Delivery
The equation in Box 2.1 shows that ḊO2 is simply proportional to the product of the three ‘Barcroft variables’. It would, therefore, appear that any given deficit in ḊO2 should cause identical degrees of cellular hypoxia regardless of whether the deficit is due to hypoxaemia, anaemia or low blood flow. We shall see below that whereas ḊO2 deficits due to anaemic and stagnant hypoxia have virtually identical consequences, ḊO2 deficits due to hypoxaemic hypoxia are very distinct and uniquely important.
Anaemic and Stagnant ḊO2 Deficits
Experimental and theoretical models show that the variables [Hb] and Q̇ are not uniquely independent variables; it is merely the product, Q̇ [Hb], which determines oxygen delivery and cellular oxygenation. For example, if haemoglobin concentration is halved and blood flow doubled, oxygen delivery and cellular oxygenation remain unchanged. This is because these variables simply determine the flux of oxygen to the tissues, and they have no other significance beyond this point.
Hypoxaemic ḊO2 Deficits
Reductions in ḊO2 due to hypoxaemia are much more impactful than if an equal ḊO2 reduction were due to anaemic or stagnant causes. This seems counterintuitive if considered in terms of Barcroft’s classification, because this focusses on oxygen delivery (bulk oxygen flux) to the tissue capillaries.
From the lung to the capillary, oxygen transport is by convection, whereas from capillary to cell/mitochondrion, oxygen transport is by diffusion. It is the PO2 in the capillary which drives the diffusion of oxygen from capillary to cell. So, the effects of hypoxaemia are twofold: not only does it reduce oxygen flux along the arterial tree (via a reduced SaO2), but it also impairs oxygen delivery beyond the tissue capillary (via a reduced PO2).
The PO2 at the cellular level is around 3–10 mmHg (0.4–1.3 kPa), and at the mitochondrion it is around 1 mmHg (0.13 kPa). The PO2 in tissue capillaries may be around 40 mmHg (5.3 kPa) and this PO2 gradient drives oxygen from capillary to mitochondrion according to Fick’s law of diffusion. Figure 2.1 shows the effect of reducing ḊO2 on the cell’s ability to take up and consume oxygen (V̇O2), and how this differs depending on whether the fall in ḊO2 is achieved via anaemic/stagnant or hypoxaemic mechanisms. It can be seen that as ḊO2 falls, V̇O2 remains constant until a critical ḊO2, ḊO2crit, is reached, below which cellular oxygen uptake and utilisation are diminished. ḊO2crit represents the oxygen delivery at which cellular hypoxia begins. In normal tissue (bold lines), cellular hypoxia is seen to begin when ḊO2 falls to 0.4 L min-1 for hypoxaemic hypoxia, whereas the cell can tolerate a lower ḊO2 if the mechanism is anaemic or stagnant. In other words, cells are more vulnerable to hypoxaemic hypoxia.
Figure 2.1 Plot of cellular O2 consumption (V̇O2) vs. bulk O2 delivery (ḊO2). Solid lines represent stagnant/anaemic hypoxia. Broken lines represent hypoxaemic hypoxia. Bold lines show normal relationship for tissue without significant barrier to O2 diffusion from capillary to cell. Feint lines represent tissue with significant diffusional resistance, as in oedema or shock. As ḊO2 falls, V̇O2 initially remains constant and satisfies the normal metabolic requirement (0.25 l min−1). When ḊO2 falls to a critical value, Ḋcrit (shown by arrows), cellular O2 consumption falls and cellular hypoxia begins. The difference in Ḋcrit between hypoxaemic and stagnant/anaemic hypoxia is shown to increase when a diffusional barrier exists).
According to Fick’s law, diffusive oxygen flux depends not only on the partial pressure gradient, but also on the distance between capillary and cell, and this may be increased in oedematous states (where the interstitium occupies a greater volume, separating capillary from cell), and in capillary de-recruitment due to shock (where, if a cell’s nearest capillary is de-recruited, its new nearest patent capillary will now be a greater distance away). This may explain why the difference between stagnant/anaemic and hypoxaemic hypoxia on cellular oxygen uptake is exaggerated in states of reduced diffusive conductance. This effect is also shown in Figure 2.1 (feint curves).
The Rate of Arterial Desaturation in Apnoea
We have seen that hypoxaemic hypoxia is of particular importance in the development of cellular hypoxia and clearly in the context of the difficult airway, the principal cause of hypoxaemia is airway obstruction. It is important to understand the mechanisms by which hypoxaemia develops, and the factors which determine the rate of this process.
As soon as apnoea (with an obstructed airway) occurs, alveolar and hence pulmonary capillary PO2 begins to fall. In apnoea, the process of gas exchange between alveolus and pulmonary capillary becomes non-linear. The rising partial pressure of carbon dioxide (PCO2) and falling pH associated with carbon dioxide accumulation continually shifts the oxygen–haemoglobin dissociation curve adding yet more non-linearity to the process of arterial desaturation. The time lag between changes in PO2 feeding through into changes in mixed venous PO2 enhances the complexity of the mathematical model further. Figure 2.2 shows the effects of six different physiological derangements on the rate of arterial desaturation in obstructed apnoea. Figure 2.2(a) shows that desaturation is exaggerated in small lung volumes (as might occur in supine anaesthetised patients). Figure 2.2(b) shows that the value of the initial alveolar oxygen concentration at the onset of apnoea is also important. Due to the various mathematical non-linearities in the system, the lower the initial alveolar oxygen tension, the greater the rate of desaturation. This has important implications for patients who have periods of partial airway obstruction (and hence diminished alveolar PO2 (PAO2)) before obstructing completely. It also underpins the value of thorough pre-oxygenation before procedures where there is a significant risk of obstructed apnoea. Figure 2.2(c) shows that while shunt diminishes the value of SaO2 at any given time during apnoea, the rate of desaturation is unaltered. Figure 2.2(d) shows that increased metabolic rates (as may occur in sepsis, or when struggling to breathe in severe airway obstruction) increases the rate of arterial desaturation, and this effect is exaggerated as desaturation proceeds. Figure 2.2(e) and (f) show how both diminished cardiac output and reduced haemoglobin concentrations increase the rate of arterial desaturation in apnoea. This is partly because haemoglobin acts as an oxygen reservoir. The effect of cardiac output is complex. So, not only does arterial hypoxaemia have a unique importance in terms of cellular hypoxia (as discussed above and in Figure 2.1), but in apnoea, anaemia and low flow states compound the reduction in SaO2 and also markedly exaggerate the reduction in oxygen delivery, which is the product of all three of these terms. The interplay of these factors is depicted in Figure 2.3.
Figure 2.2 (a) Effect of lung volume (VA in litres) on the time course of SaO2 in apnoea. (b) Effect of initial FAO2 on the time course of SaO2 in apnoea. (c) Effect of shunt fraction (fs) ranging from 0% to 15% on the time course of SaO2 in apnoea. (d) Effect of O2 consumption rate (V̇O2) ranging from 0.15 to 0.3 litre min−1 on the time course of SaO2 in apnoea. (e) Effect of haemoglobin concentration ([Hb] in g litre−1) on the time course of SaO2 in apnoea. (f) Effect of total blood volume (QT) on the time course of SaO2 in apnoea.
Figure 2.3 Reductions in both [Hb] and Q̇ as shown in (A) not only reduce oxygen delivery (B) directly (path A–B), but also indirectly via path A–C–B during apnoea because the rate of arterial desaturation during apnoea is increased by anaemia and low output. The combination of this direct and indirect effect is to produce an exaggerated reduction in oxygen delivery (B). The reduction in oxygen delivery may reduce cellular oxygen uptake (path B–D), as predicted by the solid lines in Figure 2.1. In addition, the hypoxaemic conditions (point C) contribute independently to exaggerating the reduction of cellular oxygen uptake (via path C–D) as predicted by the broken lines in Figure 2.1.
Also of note is the fact that small derangements in each of the physiological factors in Figure 2.2 combine to produce a larger overall effect on the rate of arterial desaturation. An example of this might be a ‘typical’ sick patient about to undergo induction of anaesthesia. This is shown in Figure 2.4.
Figure 2.4 Rate of arterial oxyhaemoglobin desaturation with combination of small derangements in pathophysiological variables as might be seen in a peri-operative adult. Haemoglobin = 10 g dl−1, cardiac output = 4 l min−1, initial PAO2 = 10 kPa, initial PACO2 = 8 kPa, alveolar volume = 2.0 litres, shunt fraction (fs) = 0.1).
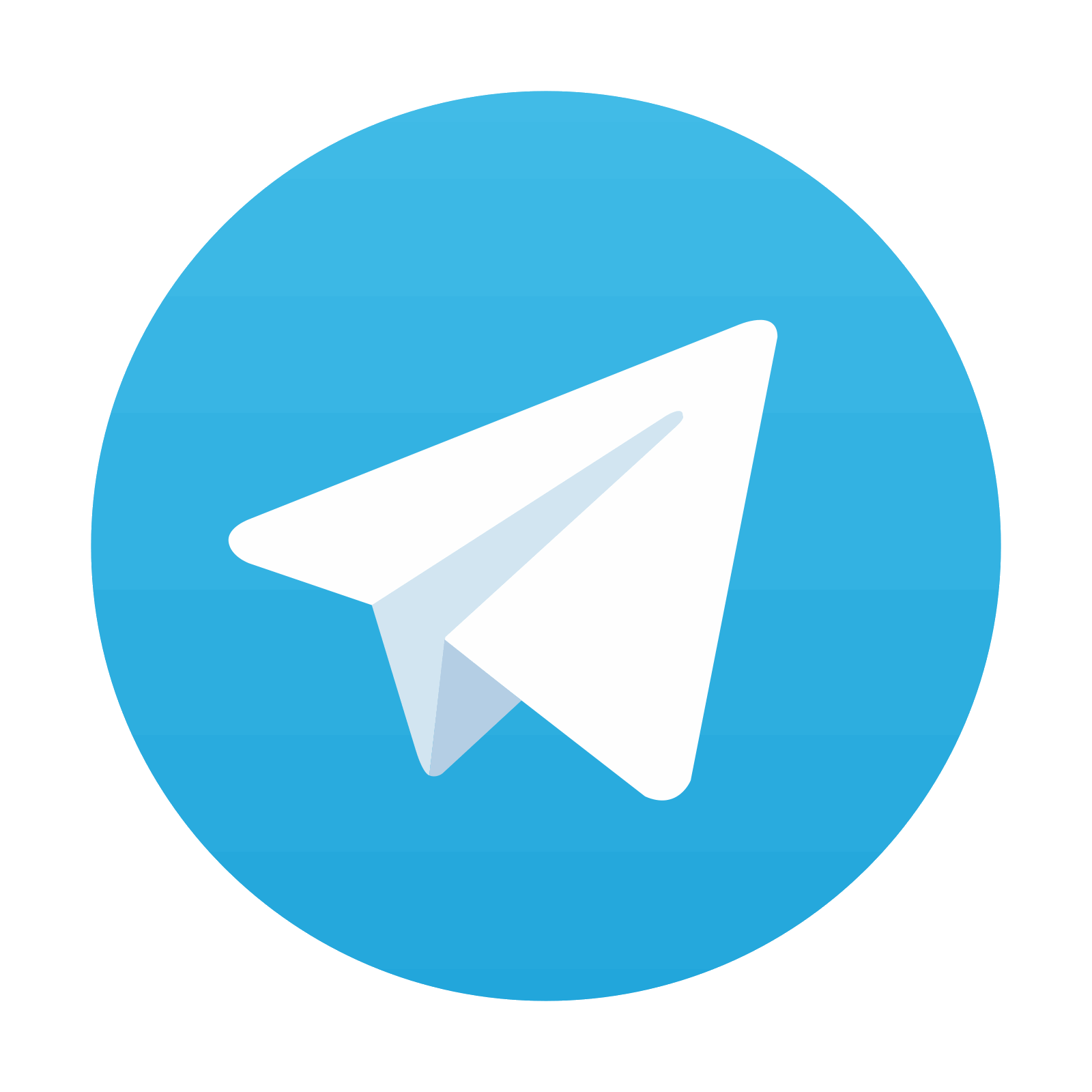
Stay updated, free articles. Join our Telegram channel
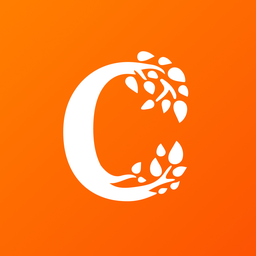
Full access? Get Clinical Tree
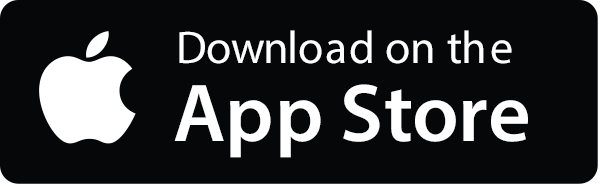
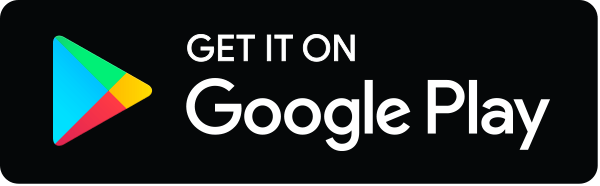
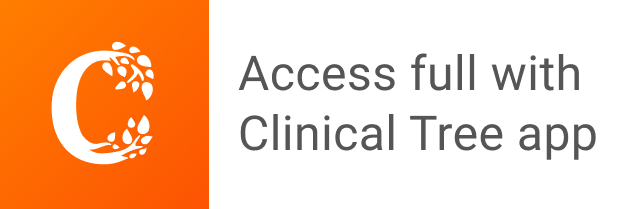