Abstract
Physiology Answers
Question 1: TFTFF
Atrial contraction accounts for 10% of ventricular filling at rest. At high heart rates this ratio increases to 40% due to limited time available for passive filling. After atrial contraction is complete, the atrial pressure starts to fall. This causes the valve to float upwards before closure. At this time the ventricular volume is maximal, which is termed the end diastolic volume (EDV).
The two main coronary arteries are the left main and right coronary arteries. The left main coronary artery supplies blood to the left side of the heart muscle (the left ventricle and left atrium). The right coronary artery supplies blood to the right ventricle, the right atrium and the SA (sinoatrial) and AV (atrioventricular) nodes, which regulate the heart rhythm.
Each cardiac myocyte is surrounded by a cell membrane called the sarcolemma and contains one nucleus. The cells are packed with mitochondria to provide the steady supply of ATP required to sustain cardiac contraction. As with skeletal muscle, cardiac myocytes contain the contractile proteins actin (thin filaments) and myosin (thick filaments), together with the regulatory proteins troponin and tropomyosin. Cardiac muscle is striated, although the pattern is not as ordered as in skeletal muscle.
Calcium has an essential role in myocardial contraction. A raised intracellular calcium concentration is the trigger that activates contraction. Diastolic relaxation is an active (ATP-dependent) process. Calcium transport out of the cytosol occurs via a sarcoplasmic reticulum Ca2+ ATPase, through sarcolemmal Na+/Ca2+ exchange.
Question 2: TTFTT
The sinoatrial (SA) node, atrioventricular (AV) node, bundle of His and other atrial centres all have inherent pacemaker activity. From the SA node, impulses spread throughout the atria to the AV node. Depolarization spreads from the AV node to the bundle of His in the interventricular septum. The bundle splits into right and left bundle branches, supplying the respective ventricles. As the left bundle branch is activated first, the depolarization proceeds from left to right and may give rise to a small negative deflection within the ECG, referred to as the Q-wave.
In LBBB, the normal direction of septal depolarization is reversed (becomes right to left), as the impulse spreads first to the RV via the right bundle branch and then to the LV via the septum. This sequence of activation extends the QRS duration to >120 ms and eliminates the normal septal Q waves in the lateral leads. The overall direction of depolarization (from right to left) produces tall R waves in the lateral leads (I, V5–6) and deep S waves in the right precordial leads (V1–3), and usually leads to left axis deviation.
In RBBB, activation of the right ventricle is delayed as depolarization has to spread across the septum from the left ventricle. The left ventricle is activated normally, meaning that the cardiac axis is not affected by RBBB and the early part of the QRS complex is unchanged.
The conduction of electrical impulses throughout the heart, and particularly in the specialized conduction system, is influenced by the autonomic nervous system. This autonomic control is most apparent at the AV node. Sympathetic activation increases conduction velocity in the AV node. Parasympathetic (vagal) activation decreases conduction velocity (negative dromotropy) at the AV node. This leads to slower depolarization of adjacent cells, and reduced velocity of conduction.
Wolff–Parkinson–White syndrome (WPW) was first described in 1930 by Louis Wolff, John Parkinson and Paul Dudley White. WPW syndrome is a combination of the presence of a congenital accessory pathway and episodes of tachyarrhythmia. The incidence rate is 0.1–3.0 per 1000. It is associated with a small risk of sudden cardiac death. It has two characteristic components: pre-excitation and accessory pathways. Pre-excitation refers to early activation of the ventricles due to impulses bypassing the AV node via an accessory pathway. Accessory pathways, also known as bypass tracts, are abnormal conduction pathways formed during cardiac development and can exist in a variety of anatomical locations, and in some patients there may be multiple pathways. In WPW the accessory pathway is often referred to as the bundle of Kent, or atrioventricular bypass tract.
Question 3: FTFFT
When considering the cardiac action potential it is important to differentiate between the nodal (pacemaker) action potential (Figure 1.3.1) and the conduction system action potential (Figure 1.3.2).
They both last approximately 250 ms and have an absolute refractory period (during which no further action potentials can be generated) and a relative refractory period (during which an action potential can be initiated by a supramaximal stimulus).
Figure 1.3.1 Nodal action potential Nodal action potential i.e. the nodal action potential is mostly to do with changes in permeability to Ca2+ and K+.
Question 4: TFFFT
Figure 1.3.2 Conduction action potential.
Automaticity refers to the ability of a cell to depolarize without stimulus. In the heart the sinoatrial (SA) node, atrioventricular (AV) node, His–Purkinje system and some cardiac myocytes contain cells that display automaticity. The SA node is the predominant pacemaker for the heart. Cells with automaticity do not have a resting membrane potential, but rather exhibit baseline drift due to Na+ leak, open T-type Ca2+ channels and a Na+/Ca2+ pump. This allows cations to move intracellularly and gradually increases the membrane potential. At –40 mV an action potential is triggered as the cell reaches the threshold potential. The SA node can be affected by the sympathetic nervous system to increase or decrease the heart rate. Sympathetic nervous input (β receptors) causes Ca2+ channels to open, causing the cells to reach the threshold potential more quickly and thereby increasing the rate at which the SA node fires, whereas parasympathetic input (muscarinic receptors) causes an increase in K+ permeability which hyperpolarizes the cell and reduces the rate of SA node firing.
Question 5: FFFTF
The events of the cardiac cycle are lengthy to describe and therefore they are often charted in a diagram. This diagram is also known as Wigger’s diagram (Figure 1.5.3). It relates the ECG trace, heart sounds, pressure waves of the left ventricle and atria, and the aorta, the left ventricular volumes and valvular activity together to give a picture of the cardiac cycle as a whole. It can be a daunting diagram, but when taken part by part it becomes much easier to understand and therefore learn (Figures 1.5.1 and 1.5.2).
Figure 1.5.1 Wigger’s diagram of ECG, heart sounds, systole, diastole and valvular activity.
Figure 1.5.2 Wigger’s diagram of pressure waves.
Figure 1.5.3 Complete Wigger’s diagram.
Question 6: FTTTF
At rest, the cardiac cycle lasts approximately 0.8 s. The diastolic period is 0.5 s, while the systolic period is 0.3 s. During tachycardia the diastolic time is shortened and becomes closer to the systolic time in duration. Many events occur during diastole.
Diastole can be divided into several phases: beginning, early, mid and late.
The start of diastole is denoted by the closure of the aortic and pulmonary valves (second heart sound). As the ventricular muscle relaxes the intraventricular pressure falls (isovolumetric relaxation) until the pressure in the ventricles is less than that in the atria, the atrioventricular valves open (marking early diastole) and the ventricles fill rapidly. This rapid filling is responsible for approximately 80% of the ventricular volume. As the ventricles fill, the pressure difference between the atria and ventricles decreases and the filling therefore slows (mid-diastole). It is during late diastole that the atria contract and force blood from the atria into the ventricles.
The coronary blood flow and therefore perfusion vary during the cardiac cycle and by region. Most of the blood flow to the coronary arteries is during diastole. Some blood flow occurs during systole, while the left ventricle is dependent on diastolic flow only due to higher pressures generated during contraction, causing compression of the overlying vessels. This means that the left ventricle is more susceptible to ischaemia.
Lusitropy refers to the relaxation of myocardium following contraction. Positive lusitropic factors include low calcium and β- adrenergic stimulation.
Question 7: TFFTF
CVP stands for central venous pressure and the waveform can be seen if a central venous catheter is transduced (Figure 1.7.1). Normal CVP in a spontaneously breathing patient is 0–8 cmH2O (not mmHg). It is useful as an estimate of right ventricular preload and to monitor adequacy of volume replacement – serial measurements are vastly more useful than single results.
Figure 1.7.1 CVP wave.
The waveform is divided into ‘waves’. There are three positive waves and two ‘descent’ or negative waves.
The ‘a wave’ is the pressure increase secondary to atrial contraction. This is increased in atrial hypertrophy and absent in atrial fibrillation. Cannon a waves are seen when the atrium contracts against a closed tricuspid valve, if the a waves are irregular it indicates complete heart block, as opposed to regular when it suggests a nodal rhythm.
The ‘c wave’ represents the increase in pressure caused by the bulging of the tricuspid valve into the right atrium during ventricular contraction.
The ‘v wave’ denotes atrial filling (closed tricuspid valve). Large v waves suggest tricuspid incompetence.
The ‘x descent’ occurs during relaxation of the atrium. The x descent can be absent in tricuspid incompetence.
The ‘y descent’ demonstrates passive ventricular filling (open tricuspid valve).
Question 8: TTTFF
The Frank–Starling law relates the fibre length at initiation of contraction to the force of myocardial contraction produced. The two are proportionally related up to a point at which the relationship fails (consider an overstretched elastic band – it doesn’t recoil with the same force as a normally stretched elastic band). This can be represented in graphical form for both normal and pathological states.
The pressure–volume relationship of the ventricles can be denoted in graphical form and can be used to demonstrate the workload of the heart. In order for a valve to open, the pressure in the chamber behind it must be greater than that of the chamber or space in front of it (i.e. the left ventricular pressure must be greater than the aortic pressure for the aortic valve to open). During the cardiac cycle there are two occasions where the pressure and volume are not inversely related. This is referred to as isovolumetric relaxation or contraction, and reflects the period of time during which the ventricles are essentially sealed with closed valves both from the atria and to the great vessels. For example, as the ventricle relaxes the pressure within will fall; however, with closed valves the volume will not change (isovolumetric relaxation)
Question 9: FFFFF
There are many methods used to calculate the cardiac output.
The simplest formula is: cardiac output (CO) = heart rate (HR) × stroke volume (SV). As stroke volume = end diastolic volume (EDV) – end systolic volume (ESV) it can also be written as: CO = HR × (EDV – ESV).
One of the most commonly used methods to calculate cardiac output is the Fick method, which relies on the Fick principle. The Fick principle may be used to calculate blood flow to individual organs and by considering the whole body as an organ, the cardiac output can be calculated. It relies on the use of a marker substance (e.g. dye, O2, CO2) and states that the blood flow through the organ in question is equal to the marker substance uptake divided by the arteriovenous difference of that substance. For cardiac output calculation, the marker used is often O2.
The Fick equation for cardiac output:

CaO2: arterial sample
Cv¯O2 : mixed venous O2 saturation from PAFC sample
All of the variables can be directly measured; however, measuring oxygen uptake is laborious and consequently an assumed value for V˙O2 is used. This is called the Fick determination and the assumed value used is 250 ml.min−1 (125 ml.min−1.m−2). Another assumption made when using this calculation is that there are no intracardiac or intrapulmonary shunts and therefore that the pulmonary blood flow is equivalent to the systemic blood flow.
Question 10: TFFTF
Preload refers to the ventricular wall tensions before the start of systole (at the end of diastole) and is therefore related to the length of the cardiac muscle fibre at this time. As such it is described by the Frank–Starling relationship. It is associated with the end diastolic volume, but its clinical measurement is usually taken as the CVP (if looking at the right side of the heart) or the pulmonary capillary wedge pressure (left side of the heart).
Afterload is the ventricular wall tension required to eject blood during systole. It is the work required to overcome resistance beyond the ventricle and is often therefore considered clinically in terms of systemic vascular resistance (SVR). Anything that increases resistance in the arterial circulation will increase afterload. An increased afterload means increased myocardial work, decreased stroke volume and increased end systolic volume.
Contractility refers to the ability of myocardial muscle fibres to shorten (contract). It is affected by extrinsic factors (preload and afterload) and intrinsic factors (autonomic activity and catecholamines). Drugs affecting contractility are said to have an inotropic effect; this may be positive or negative. It is difficult to measure contractility and therefore indirect methods are used, such as stroke volume, cardiac output and ratio of left end diastolic pressure to left end diastolic volume.
Question 11: FTFTF
The normal range of heart rate varies by individual but is stated as 60–100 bpm. Tonic activity from the sympathetic outflow to the heart is mediated via T1–5 (usually dominant) and parasympathetic tone via the vagus nerve. The balance of sympathetic and parasympathetic tone determines heart rate. The heart rate can also be affected by hormonal mechanisms including adrenaline (via β1 receptors). There are many afferent inputs to the medulla that influence the balance of autonomic tone and these are integrated by the nucleus tractus solitarius (NTS) and the rostral ventrolateral medulla (RVLM).
The arterial supply is via the right coronary artery (RCA) from the anterior aortic sinus and the left coronary artery (LCA) from the posterior aortic sinus. The RCA supplies the right side of the heart, the sinoatrial node and (in most) the atrioventricular node. The LCA supplies the left side of the heart and the interventricular septum. The RCA is the dominant vessel in 50% of people and in 30% the RCA and LCA have equal dominance.
Coronary blood flow is autoregulated (60–80 mmHg) via many mechanisms: myogenic, metabolic, endothelial vasoactive substances and hormones (e.g. ANP is a vasodilator and ADH and angiotensin II are vasoconstrictors)
Question 12: FFTTT
The Valsalva manoeuvre is the action of forced expiration against a closed glottis after full inspiration. A pressure of 40 mmHg should be achieved and held for 10 s. Four phases occur during the manoeuvre where changes in the blood pressure (BP) and heart rate are observed.
Phase 1: Increase in intrathoracic pressure causes an initial increase in venous return, resulting in increased BP and lowering of the heart rate.
Phase 2: The sustained high intrathoracic pressure reduces venous return and BP falls. Baroreceptors sense a reduction in stretch and the baroreceptor reflex is stimulated to restore normotension.
Phase 3: At the end of the manoeuvre, the release of intrathoracic pressure creates a large empty venous reservoir. The venous return pools in the pulmonary vessels which reduces preload and causes the BP to fall.
Phase 4: The baroreceptors respond to the drop in BP by reducing their inhibitory effect on the pressor centre, causing vasoconstriction and tachycardia. Once venous return is restored, there is a compensatory overshoot resulting in hypertension and a baroreceptor-mediated bradycardia before both parameters eventually return to normal.
The Valsalva manoeuvre can be used to assess autonomic function or to terminate a supraventricular tachycardia. The manoeuvre also increases the intensity of hypertrophic cardiomyopathy murmurs and decreases the intensity of most other murmurs.
In autonomic neuropathy there is an exaggerated drop in BP during phase 2 with no overshoot or bradycardia in phase 4, this is due to diminished baroreceptor reflexes. A square-wave response is seen in congestive cardiac failure, tamponade and valvular disease. The square wave response occurs when the CVP is markedly raised and is characterized by a rise instead of a drop in BP during phase 2.
The BP fall in phase 2 will be exaggerated in hypovolaemic patients and those with sympathetic block (e.g. β-blockers and regional anaesthesia).
Question 13: FFFTF
The blood pressure is tightly controlled by both short- and long-term regulatory mechanisms. Short-term blood pressure regulation is largely mediated by arterial and cardiac baroreceptors and the vasomotor centre, which alters the balance between sympathetic and parasympathetic nervous systems. Long-term blood pressure control is mediated by neurohumoral, renal, metabolic and genetic factors.
Baroreceptors are stretch receptors; they are classified into high- and low-pressure receptors. High-pressure receptors are located within the aortic arch and the carotid sinus. They are responsible for rapid, short-term blood pressure control. Low-pressure baroreceptors are found within the heart, in large systemic veins and the pulmonary vasculature. These receptors bring about changes in blood volume and are responsible for slower changes in blood pressure.
The carotid sinus is situated at a dilatation at the bifurcation of the internal carotid. Carotid sinus baroreceptors discharge impulses back to the vasomotor centre via a branch of the glossopharyngeal nerve (IX). The baroreceptors in the aortic arch transmit impulses via the vagus nerve (X).
The vasomotor centres are located in the medulla. The pressor area is located in the ventrolateral medulla and the depressor area (cardioinhibitory centre) in the ventromedial medulla. In hypotension, there is decreased baroreceptor stretch, the pressor area is stimulated (via glossopharyngeal and vagus afferents) and efferent signals to sympathetic preganglionic neurones in the spinal cord result in vasoconstriction, tachycardia and increased blood pressure. In hypertension, the resultant increase in baroreceptor discharge results in the stimulation of the depressor area and inhibition of the vasomotor centre, causing a reduction in sympathetic tone and increased vagal activity.
Higher centres, such as the hypothalamus, cerebral cortex and limbic system, exert some influence on the vasomotor centres. The nucleus tractus solitarius (NTS) is the sensory nucleus for both the vagus and the glossopharyngeal nerves. Afferent signals from chemo- and baroreceptors travel via the NTS and provide inhibitory signals to the vasomotor centre.
The Bainbridge reflex is an increase in heart rate due to an increase in central venous pressure.
Question 14: FTFFF
The normal left ventricular end diastolic volume (LVEDV) is 120–150 ml in an adult. The LVEDV is often used as a measure of preload. Preload is the LV wall tension at the end of diastole and reflects maximal length of the ventricular sarcomeres just prior to contraction. In clinical practice, surrogates for preload measurement include LVEDV (measured by echocardiogram), pulmonary artery occlusion pressure when studying the LV, and central venous pressure when studying the right ventricle.
The Frank–Starling law of the heart states that the stroke volume of the heart increases in response to an increase in the volume of blood filling the heart (LVEDV) when all other factors remain constant. A normal stroke volume is 70–80 ml. Stroke volume is calculated by end diastolic volume (EDV) minus end systolic volume (ESV). Ejection fraction is the percentage of ventricular volume that is ejected from the ventricle during systolic contraction. A normal ejection fraction is 55–70% and is a measure of contractility. At rest a normal LVESV is 50–70 ml.
The LVEDV (or preload) is determined by venous return, heart rate, atrial contraction and ventricular compliance. Poorly compliant ventricles, seen post myocardial infarction and in ventricular hypertrophy for example, take longer to fill, relying more upon the atrial ‘kick’ for filling.
In systolic heart failure, the ejection fraction will be decreased and the LVEDV will be increased. In diastolic failure the ejection fraction is normal and the LVEDV will be normal to low since the ventricle is non-compliant and difficult to fill in diastole.
During strenuous exercise, oxygen consumption may increase up to 4000 ml.min−1. Heart rate and stroke volume increase to about 90% of their maximum values in order to increase cardiac output. Venous return increases due to the pumping action of the muscles. Locally released mediators cause vasodilation in the contracting muscles resulting in decreased afterload. The increased inotropy and chronotropy in the heart results in a reduction in LVESV (due to more forceful ventricular contractions) and there is an increased LVEDV due to increased venous return. Trained athletes have lower heart rates, greater LVEDV and greater stroke volumes at rest.
Question 15: TFFTF
The cardiovascular system is closely controlled by both systemic and local control mechanisms. Systemic regulation is achieved by neural regulatory mechanisms, using baroreceptors and the vasomotor centre in the medulla to adjust sympathetic tone and vessel calibre, and the neurohumoral system. Local regulatory mechanisms also exist and allow tissues to autoregulate their own blood flow; examples of this occur in the kidney, abdominal mesentery, skeletal muscle, brain, liver and myocardium.
Autoregulation is thought to occur as a result of the intrinsic contractile response of smooth muscle to stretch (myogenic theory) and due to the accumulation of vasodilator substances in active tissues (metabolic theory). When blood flow decreases, these vasodilator substances accumulate, causing vessel dilatation, thus improving blood flow.
Metabolic changes in the tissues that produce vasodilation include decreases in oxygen tension and pH. Increases in carbon dioxide tension, lactate, osmolality and K+ also dilate vessels. A rise in temperature exerts a direct vasodilator effect. Adenosine has a vasodilatory effect in cardiac muscle, but not in skeletal muscle.
Injured vessels constrict strongly. A drop in tissue temperature causes vasoconstriction; this process is important in temperature regulation.
Endothelial cells secrete a number of vasoactive substances, including prostaglandins, thromboxanes, nitric oxide and endothelins. Prostacyclin is produced by endothelial cells. It inhibits platelet aggregation and promotes vasodilation. Thromboxane A2 opposes the actions of prostacyclin on platelets and promotes vasoconstriction. Nitric oxide plays a key role in vasodilation. Endothelin-1 is a local, paracrine regulator of vascular tone and causes vasoconstriction.
Other circulating vasodilator hormones include kinins, VIP, substance P and atrial natriuretic peptide (ANP). Circulating vasoconstrictor hormones include vasopressin, noradrenaline, adrenaline and angiotensin II.
Question 16: TTFFF
Question 17: FTFFT
Reynold’s number (NR) is a dimensionless number and predicts whether the flow is laminar or turbulent within a tube. If NR <2000, the flow is usually laminar. If NR>4000, the flow is turbulent. For NR between 2000 and 4000, the flow could either be laminar or turbulent.

where ρ = density, v = mean velocity, D = diameter of the tube, η = viscosity.
Thus the equation indicates that large diameter, high velocity and low viscosity predispose to a higher NR and hence a turbulent flow.
Question 18: TFTTT
The lymphatic system serves to collect the exuded plasma and its constituents from the interstitial space. The protein content of lymphatic fluid is lower than in plasma (1.5 versus 6%). The lymph vessels are blind-ended, thin-walled and lack tight junctions in their endothelium. They have one-way valves and drain via a system of lymphatic vessels, eventually entering the subclavian veins at their junctions with the respective internal jugular veins.
The thoracic duct is the largest lymphatic vessel and transports up to 4 litres of lymph per day. In adults, the thoracic duct is typically 38–45 cm in length and usually starts from the level of the second lumbar vertebra, extending to the root of the neck. It drains into the systemic circulation at the left subclavian vein and collects most of the lymph in the body, other than from the right thorax, arm, head and neck, which are drained by the right lymphatic duct.
Lymphatic flow is aided by skeletal muscle contractions, negative intrathoracic pressure (i.e. by the patient breathing), and pressure created by the blood flow from adjacent arteries and veins.
Question 19: TFTTF
The arterioles are the major site of the resistance to blood flow, and small changes in their calibre cause large changes in the total peripheral resistance. The capillaries range in diameter from 5–10 μm. The capillary wall is made of single layer of endothelium.
Blood is a non-Newtonian fluid; its viscosity is affected by changes in temperature, which can affect flow.
Precapillary sphincters are not innervated and they respond to local or circulating mediators.
Question 20: TFTFF
Question 21: FTFTT
Any lung volume that encompasses the residual volume cannot be measured with spirometry, as by definition, it is the volume of air remaining in the lungs after maximal expiration. Therefore, spirometry can measure vital capacity, tidal volume, inspiratory reserve volume and expiratory reserve volume. Functional residual capacity (FRC) is the volume of air remaining in the lungs after a normal expiratory breath. It is measured using the nitrogen washout technique, helium dilution technique or body plethysmography. Total lung capacity (TLC), the total volume of air in the lungs after maximal inspiration, is measured by body plethysmography.
Question 22: FTFTT
Compliance is defined as the change in volume per unit change in transpulmonary pressure. Therefore the units are ml.cmH2O−1, and compliance is determined by the slope of the pressure–volume curve. Normal values for both static and dynamic compliance are 60–100 ml.cmH2O–1. Static compliance is always greater than dynamic compliance due to the variation in time constants in different areas of the lung (i.e. how quickly a lung compartment can react to an alteration in pressure). Compliance is best at the FRC and is influenced by pathological and physiological factors. Physiological factors which decrease compliance include supine posture, pregnancy and extremes of age. Pathological factors which decrease compliance can be classified into extrapulmonary, which include the chest wall (kyphoscoliosis), and intrapulmonary, which include pulmonary fibrosis, ARDS and atelectasis. The only pathological factor that increases the lung compliance is emphysema.
Question 23: FFFFT
Respiratory dead space (physiological dead space) is defined as the volume of inspired gas that does not take part in gas exchange. It is determined by the totals of the anatomical dead space (calculated by Fowler’s method) and the alveolar dead space (determined by the Bohr equation).
Physiological dead space = anatomical dead space + alveolar dead space
The V/Q ratio is therefore infinity. The respiratory dead space is decreased by general anaesthesia due to increased shunt. As dead space increases, alveolar ventilation decreases, therefore paCO2 will increase.
The V/Q ratio at the apices of the lungs is relatively higher, as they are more well-ventilated than perfused compared to the lung bases, where ventilation and perfusion are highest. Thus, alveolar dead space in the apices is increased.
Question 24: TTTTT
The work of breathing is the work required by the respiratory muscles in order to overcome the mechanical impedance to respiration caused by the lung, chest wall and abdominal contents during breathing. It is the sum of the work required to overcome both elastic properties and flow resistance, and is determined by the area contained inside a pressure–volume curve. The elastic forces are stored as potential energy during inspiration.
Although expiration is usually passive, i.e. does not require energy expenditure (utilizes the potential energy stored in the respiratory muscle during inspiration), during forced expiration, or in a patient with airway disease, additional expiratory mechanical work may be required, even in tidal breathing. The frictional forces of expiration are due to airway resistance. For example, in asthma, there is an increase in flow-resistive work as a result of the airway narrowing. Here, the elastic energy stored during inspiration will not produce enough airflow during expiration and the expiratory muscles must cope with an increased load and perform extra work.
Any work to overcome non-elastic forces is lost as heat. In a ventilated patient, the ventilator tubing and the endotracheal tube increase the airway resistance, therefore increasing the work of breathing.
Question 25: TFTFT
The FRC is the volume of air left in the lungs at the end of a normal tidal breath. It is approximately 30 ml.kg−1 and effectively provides an oxygen reservoir as a buffer against injury. Here, gas exchange continues to occur throughout the respiratory cycle. It prevents airway collapse and atelectasis, therefore pulmonary vascular resistance is lowest at FRC. The closing capacity is the volume of the lung at the point when small airways begin to collapse during expiration. It is the sum of the residual volume and the closing volume. Closing capacity increases with age and so encroaches upon the FRC. This means that there is an increased ventilation/perfusion (V/Q) mismatch.
FRC decreases under general anaesthesia due to the loss of muscle tone reducing the bucket handle action of the rib cage.
FRC typically reduces by approximately 18–20% during pregnancy, due to compression of the diaphragm by the gravid uterus. In the pregnant woman, in order to overcome the reduction in FRC, total lung capacity, expiratory reserve volume and tidal volume increase by 30–40%. Minute ventilation increases, giving an increase in pulmonary ventilation necessary to meet the increase in oxygen requirement.
Question 26: FFTTF
The main muscle of respiration is the diaphragm, which during normal respiration is responsible for 75% of the air that is drawn into the lungs. Two-thirds of diaphragmatic muscle fibres are of the slow twitch variety so as to reduce its fatigability. The lungs and the thoracic cage are the two main mechanical components of the respiratory system.
A pressure gradient exists across the surface of the lungs known as the transpulmonary pressure, which is equal to the difference between the airway pressure and the pressure at the lung surface (intrapleural pressure). The natural tendency of the lungs is to collapse, while that of the rib cage is to spring outwards. This produces a pressure differential between the two pleural layers, parietal and visceral – the intrapleural pressure.
The accessory muscles include the sternocleidomastoids, the scalene muscles and strap muscles of the neck. While these are relatively inactive in normal respiration, they do serve to stabilize the upper rib cage and prevent in-drawing of the ribs.
Compliance is defined as the change in volume per unit change in pressure.
Question 27: TTTFF
Type II alveolar epithelial cells secrete surfactant. An important constituent of surfactant is dipalmitoylphosphatidylcholine (DPPC), synthesized from fatty acids. Surfactant synthesis and its turnover are rapid, production begins relatively late in gestation; hence its deficiency before 32–34 weeks’ gestation can predispose to respiratory distress syndrome. Surfactant serves to reduce surface tension and, in so doing, it improves the pulmonary compliance and reduces the tendency of the alveoli to draw in fluid from interstitial capillaries, keeping the alveoli relatively dry.
Hysteresis (defined as the change in the measurement value depending on whether the value is increasing or decreasing) of the pressure–volume loop is the result of the energy expended as heat in the respiratory cycle. Surfactant reduces not only the work of breathing, but also the heat expended and therefore results in a reduction in the volume of the hysteresis area of the pressure–volume curve.
Surfactant also serves to stabilize the smaller alveoli, as these are most prone to collapse due to surface tension. In stabilizing the smaller alveoli, surfactant makes them less likely to collapse and empty their contents to adjacent larger alveoli, but has no effect on the stability of larger alveoli per se.
Question 28: FTFFT
From apex to base, both ventilation and perfusion increase in the upright lung; however, the increase in perfusion is much greater. This results in a ventilation/perfusion ratio (V/Q ratio) that is greater at the apex than it is at the base, being approximately equal to 1 at the level of the third rib.
The difference in partial pressure between the apex and base of the lung is greater for oxygen; this is because the uptake of oxygen at the apex tends to be poor due to the low blood flow at rest. This increases in exercise when blood flow throughout the lung is more uniform. Carbon dioxide partial pressures are less affected by blood flow and influenced more by ventilation; the difference in CO2 output between apex and base is much less marked.
While hypercarbia can be compensated for by an increase in ventilation (in order to reduce the partial pressure of carbon dioxide in the alveoli and in turn in the blood), hypoxaemia cannot be corrected in this way. The main way to increase the partial pressure of oxygen in the bloodstream is to increase the fraction of inspired oxygen. Pulmonary emboli obstruct blood flow to the lungs, i.e. perfusion is reduced. Ventilation throughout the lung remains constant, thus the ventilation/perfusion ratio is increased.
Question 29: TTFTF
The balance between oxygen supply (alveolar ventilation) and demand (removal by pulmonary capillaries to supply the tissues) determines the pO2 of alveolar gas. At rest, the removal of oxygen from the lungs (i.e. tissue oxygen usage) is relatively constant and so alveolar ventilation becomes the main determinant of pAO2. Hypoventilation results in inefficient gas exchange and so pO2 falls while PCO2 is seen to rise, in turn causing an increase in paCO2.
The respiratory quotient is used in the alveolar gas equation, which uses the composition of inspired gas and the respiratory quotient to calculate alveolar pO2:
(Where pIO2 is the partial pressure of inspired oxygen, F is a small correction factor and R is the respiratory quotient, which normally = 0.8.) R is calculated by CO2 production/O2 consumption (200/250 = 0.8), and is dependent on the type of meal, i.e increased in high carbohydrate meal.
paCO2 takes longer to reach equilibrium as compared with pAO2 because body CO2 stores are much greater than O2 stores and so reaching a steady state value takes longer.
Shunt refers to regions of lung that are perfused but where ventilation is deficient. This does occur in health but can be exacerbated in disease and also partly explains why paO2 is less than pAO2.
Shunts can be classified into:
1. Physiological (5%): intrapulmonary (bronchial vein) and extrapulmonary (thebesian veins)
2. Pathological: intrapulmonary (ARDS) and extrapulmonary (PFO and PDA).
Question 30: TTFTF
This question relates to changes in blood flow occurring in the lung from the apex to the base described by West as Zones 1–3. Where Pv = venous pressure, Pa = arterial pressure and PA = alveolar pressure. In Zone 1: PA>Pa>Pv. This situation does not arise under normal conditions, but can occur with positive pressure ventilation or, alternatively, when arterial blood flow is compromized (massive haemorrhage).
In Zone 2: Pa>PA>Pv and, so, it is here that the difference in arterial and alveolar pressures determines blood flow. While the alveolar pressure is relatively constant throughout the lung, the arterial pressure and therefore the pressure driving blood flow increase down the zone. This effect observed in Zone 2 is referred to as the Starling resistor, sluice or waterfall effect.
In Zone 3, Pa>Pv>PA, i.e. the venous pressure now exceeds the alveolar pressure and so it is here that the arteriovenous pressure difference determines blood flow in the usual way. Like Zone 2, an increase in blood flow is also observed down this zone, attributed to increased capillary dilatation moving downward through the zone.
Question 31: TFFTF
IPPV results in some unfavourable pulmonary physiological effects such as: maldistribution of gas, progressive atelectasis with a reduced FRC, increased ventilation/perfusion (V/Q) mismatch, decreased compliance and a reduction in surfactant.
In spontaneously breathing patients, both ventilation and perfusion are preferentially distributed to the dependent zones of the lungs. With IPPV, preferential ventilation of the non-dependent regions occurs (due to lower resistance to flow), resulting in increased V/Q mismatch.
IPPV in the supine position leads to decreased FRC, due in part to decreased lung volume from cephalad displacement by the diaphragm and abdominal contents. The loss of lung volume contributes to atelectasis and reduced compliance.
Pulmonary hypoperfusion from IPPV, especially in the non-dependent regions with maldistribution of gas, leads to increased alveolar dead space ventilation. Dead space ventilation increases with rapid respiratory rates, age and lung pathology.
Pulmonary vascular resistance (PVR) is increased at very high lung volumes due to stretch of the pulmonary vessels and low lung volumes due to compression of the pulmonary vessels. PVR is lowest at FRC.
PEEP is used to reduce airways resistance (wider calibre airways at higher FRC), increase FRC and to prevent or reverse lung collapse. PEEP increases anatomical dead space.
Most cardiovascular side effects of IPPV correlate with mean intrathoracic pressure and reduced venous return.
Humoral effects of IPPV include an increase in ADH, renin–angiotensin and atrial natriuretic peptide, leading to overall retention of sodium and water.
Question 32: TFFTF
Barometric pressure decreases exponentially as the distance from the Earth’s surface increases. The partial pressure of oxygen decreases at increasing altitude, but the composition of air (e.g. the FiO2) does not change.
The compensatory changes to high altitude can be divided into acute and chronic. The most important acute physiological response to high altitude is hyperventilation. Hyperventilation is triggered by hypoxic stimulation of the peripheral chemoreceptors. The resultant hypocarbia and respiratory alkalosis lead to a leftward shift of the oxygen dissociation curve. The respiratory alkalosis is slowly corrected by renal compensation. The respiratory alkalosis stimulates increased 2,3-DPG production and eventually causes the OHDC to move towards the right, thus improving oxygen delivery to the tissues. Pulmonary vasoconstriction also occurs in response to alveolar hypoxia. This increases the pulmonary arterial pressures and increases the strain on the right heart.
One of the body’s chronic responses to high altitude includes polycythaemia due to increased erythropoietin release from the kidney. Although the additional oxygen-carrying capacity is beneficial, polycythaemia also causes increased blood viscosity, which negates some of its beneficial effects. Chronic hypoxic pulmonary vasoconstriction causes right ventricular hypertrophy. Myoglobin concentration increases and peripheral capillary proliferation occurs.
Question 33: TFFTT
The lung is responsible for several important non-respiratory functions. These functions include:
Vascular reservoir: The pulmonary circulation has extremely distensible vasculature; this enables it to cope with large fluxes in venous return, particularly during exercise and postural changes. Changes in pulmonary vascular volume are also influenced by the sympathetic nervous system. 70–100 ml of blood is contained within the pulmonary capillaries, which takes part in gas exchange.
Filter for blood: Clots, fibrin clumps and air bubbles are all filtered from peripheral venous blood by the lungs, preventing them from entering the systemic circulation. Pulmonary endothelium also produces substances (fibrinolysin activator) that break down blood clots in the pulmonary circulation.
Immune function: The mucociliary escalator is the first of line defence against inhaled physical substances. As well as a physical barrier, the lungs provide an immune function that is mediated by pulmonary alveolar macrophages and a variety of immune mediators. Immunoglobulins (IgA) are also present in the bronchial secretions.
Endocrine and metabolic function: The pulmonary endothelium selectively takes up norepinephrine and serotonin from circulating blood while sparing histamine, dopamine and epinephrine; 30% of norepinephrine and 98% of serotonin is removed by this process. Angiotensin-converting enzyme (ACE) is present in large quantities in the pulmonary vascular endothelium. Angiotensin I is converted to the vasoactive peptide angiotensin II by ACE.
Drug metabolism: The lung is a small but important extrahepatic site for drug metabolism by the cytochrome P450 system. The system is easily saturated, but unlike the hepatocytes, cannot be induced.
Major role in acid/base balance
Question 34: FTFFF
Haemoglobin (Hb) is the protein responsible for carrying almost all of the oxygen within the blood; only a small percentage of oxygen is dissolved in solution. Hb is composed of four subunits, each consisting of a haem group and a globin chain. The haem group itself is composed of a protoporphyrin ring containing iron in its ferrous state (Fe2+) at the centre. The Hb molecule has four binding sites for oxygen (the iron atoms in the four haem groups) and therefore is able to cooperatively and reversibly bind up to four oxygen molecules. Oxygen can only bind to iron in the ferrous state. Methaemoglobin contains iron in the ferric/oxidized state (Fe3+) and hence cannot carry oxygen.
Adult haemoglobin (HbA) has two α and two β globin chains, whereas fetal haemoglobin (HbF) contains two γ and two α chains. When fully saturated, each gram of HbA carries 1.306 ml of oxygen. However, when fully saturated, HbF will carry more, at 1.312 ml.g−1. This allows placental transfer of oxygen from mother to fetus.
Increases in 2,3-DPG, hydrogen ion and carbon dioxide concentrations all reduce the access of oxygen to the haem portion as a result of a conformational change within the Hb molecule (by manipulating bonds between amino acids). This results in decreased affinity of Hb for oxygen. 2,3-DPG is a by-product of glycolysis, specifically reducing oxygen affinity by binding to the β chains. Therefore, more oxygen may be offloaded to the tissues, a survival benefit.
The reduction in oxygen–Hb affinity in the presence of an increased carbon dioxide or hydrogen ion concentration, or an increase in temperature, is known as the Bohr effect. The Haldane effect has no bearing on oxygen binding, but refers to the increased ability of deoxygenated blood to carry carbon dioxide. Conversely, oxygenated blood has a reduced capacity for carbon dioxide.
Question 35: TFFFF
Minute ventilation is influenced by information from both central and peripheral chemoreceptors, which relay signals to the medullary respiratory centre.
Central chemoreceptors are situated in the ventrolateral medulla near the respiratory centre and are stimulated predominantly by a rise in hydrogen ion concentration within the nearby cerebrospinal fluid (CSF). However, circulating H+ ions are unable to cross the tight junctions of the blood–brain barrier (BBB), and therefore, central chemoreceptors are not influenced by plasma pH. Their predominant mode of action is to increase ventilation in response to hypercarbia. Unlike H+ ions, circulating CO2, which is raised in respiratory acidosis, is able to cross the BBB. Within the CSF, the excess CO2 combines with H2O to form carbonic acid. This dissociates to form H+ ions, which then stimulate the central chemoreceptors. Central chemoreceptors are unaffected by hypoxia.

Hypoxia is the predominant stimulus for the peripheral chemoreceptors. These are situated in the carotid and aortic bodies and relay information to the medulla via the glossopharyngeal and vagus nerves, respectively. These specialized receptors are richly invested with capillary networks and derive their oxygen needs from dissolved oxygen; hence they are very sensitive to fluctuations in low oxygen tensions. Do not confuse this with conditions where the content of oxygen is decreased, e.g. anaemia (where oxygen tension may be normal, despite the low content). The carotid and aortic bodies also respond to the carbon dioxide tension and plasma pH, but these are less influential than hypoxia.
Control of ventilation is mainly influenced by the central chemoreceptors, which are very sensitive to small fluctuations in CSF pH. Significant degrees of hypoxia are required to stimulate the peripheral receptors. However, their role becomes more significant at altitude or in cases of chronic carbon dioxide retention, where there is blunting of the central receptor response.
An increase in body temperature does stimulate ventilation, but this is via the respiratory centre directly, not the chemoreceptors.
Question 36: TTFTF
Haemoglobin is the primary non-bicarbonate buffer in the extracellular fluid. It is a weak acid and acts as a buffer by accepting hydrogen ions (through its histidine residue). This is another example of the action of carbonic anhydrase; during acidic conditions, plasma carbon dioxide diffuses into the erythrocyte where it combines with water to form carbonic acid in a reaction catalyzed by erythrocyte carbonic anhydrase. Carbonic acid then dissociates to form hydrogen ions and bicarbonate. H+ binds to histidine residues on the globulin chains. Bicarbonate then diffuses out of the erythrocytes into the plasma. To maintain electrical neutrality of the cell, chloride ions diffuse into the erythrocytes from the plasma (‘chloride shift’) (Hamburger effect).
Deoxygenated haemoglobin is a more powerful buffer than its oxygenated counterpart. It has a pKa of 8.1 versus 6.8, hence it is less acidic and acts as a more effective base to accept the protonated H+. Deoxygenated blood is therefore a more effective carrier of carbon dioxide at the tissue level. The converse is seen at the lungs, where oxygenated blood is less able to bind CO2, which is ‘offloaded’ and eliminated from the body. This is known as the Haldane effect. Although there is a significant difference in the amount of CO2 carried in arterial and venous blood, haemoglobin is such an effective buffering system that there is only a small difference between the pH of arterial and venous blood.
Question 37: TTTFF
Oxygen therapy should be administered and prescribed appropriately; under certain conditions it can be toxic, with a host of deleterious side effects. Oxygen toxicity is caused by exposure to oxygen at partial pressures greater than those to which the body is generally accustomed. This occurs in three principal settings: underwater diving, hyperbaric oxygen chambers and supplemental oxygen therapy. Toxicity is related to free radical production. These reactive oxygen species can damage cell structures and result in oxidative stress to certain tissues.
The goal of supplemental oxygen therapy is usually to use the lowest FiO2 possible for the minimum time in order to ensure adequate tissue oxygenation. With regards to duration of oxygen therapy and risk of toxicity, FiO2 1.0 should be limited to a period of less than 12 hours, FiO2 0.8 to less than 24 hours and FiO2 0.6 to less than 36 hours.
Pulmonary effects of toxicity are more common, and occur with exposure to FiO2 of 0.5 or more at atmospheric pressure. Symptoms result from airway and pulmonary inflammation and include a ‘tickle’ leading to frequent coughing. If oxygen is not discontinued, this can progress to dyspnoea, substernal discomfort, pulmonary oedema and finally ARDS due to diffuse alveolar damage. Historically, this was known as the Lorrain–Smith effect. Breathing 100% oxygen eventually leads to absorption atelectasis.
The neonate is especially at risk following exposure to high inspired oxygen concentrations. Retrolental fibroplasia is strongly associated with hyperoxia in the developing infant (although the mechanism differs). Preterm newborns are known to be at higher risk for bronchopulmonary dysplasia. Others at higher risk include patients on mechanical ventilation (with FiO2 >0.5) and those on particular chemotherapy agents, such as bleomycin.
Exposure to partial pressures of oxygen above 160 kPa, i.e. supra-atmospheric, for as little as a few minutes is associated with central nervous system toxicity. Therefore, those most at risk are underwater divers and patients having hyperbaric oxygen therapy. Toxicity is characterized by perioral twitching, tinnitus, confusion, seizures and drowsiness (Paul Bert effect). Tremors are usually associated with hypercarbia and renal failure is not known to occur as a result of hyperoxia.
Question 38: FFTTT
Acute respiratory failure is a common reason for admission to critical care units. It is often secondary to lower respiratory tract infection, but there are other important causes.
The concept of a critical care unit, with respiratory support, arose from the polio epidemics of the 1950s. The polio virus can damage motor neurones, including those that supply intercostal nerves. Polio patients with respiratory failure were originally treated with negative pressure ventilators (‘iron lungs’). Poliomyelitis is now almost completely eradicated in the UK due to vaccination.
Tetanus results from infection with tetanus toxoid produced by Clostridium tetani. This potent neurotoxin affects striated, skeletal muscle and causes tetanic contractions such as trismus and opisthotonus. Acute respiratory failure is not a feature.
Guillain–Barré syndrome is an acute, ascending polyneuropathy, which has a sudden onset and affects the peripheral nervous system. This can involve paralysis of the diaphragm, subsequent respiratory failure, and potentially a requirement for intubation and ventilation.
Hypersensitivity pneumonitis is an alternative term for extrinsic allergic alveolitis. It may be triggered by a range of allergens (including plastics, hay and pigeon feathers), which cause an acute hypersensitivity reaction similar to asthma, but which may progress to chronic pulmonary fibrosis.
Aspirin in overdose stimulates the respiratory centre in the medulla, causing hyperventilation and respiratory alkalosis. If a patient with known aspirin overdose shows signs of respiratory failure, this should raise the suspicion of ingestion of other drugs.
Question 39: TFFFT
Acute lung injury (ALI) is a common condition that is characterized by acute severe hypoxia that is not due to left atrial hypertension. The term ALI encompasses a continuum of clinical and radiographic pulmonary changes, with acute respiratory distress syndrome (ARDS) being at the most severe end of the spectrum. ALI is still associated with a high mortality and, in essence, is the presence of non-cardiogenic pulmonary oedema and respiratory failure in the critically ill patient.
Correct diagnosis of ALI is essential as other causes of hypoxaemia may be present that are more easily treated. The definition in current use was described in 1994 at the American–European Consensus Conference and was created in order to make it easier to classify and study the diseases epidemiologically. Strengths of these criteria are that they are clinically relevant and easy to use. Weaknesses are that they are non-specific and subject to interpretation.
Diagnostic criteria are: acute onset, bilateral infiltrates on chest X-ray consistent with oedema (air bronchograms may be seen, but these are not diagnostic), pulmonary artery wedge pressure (PAWP) <18 mmHg or clinical absence of left atrial hypertension and hypoxaemia with a paO2 /FiO2 <40 (if paO2/FiO2 <27, this indicates ARDS).
Question 40: TTTTF
Respiratory failure occurs when there is inadequate gas exchange by the respiratory system. It is diagnosed by a paO2 <8.0 kPa in the arterial blood. p may be low or normal (type 1 respiratory failure) or elevated (type 2 respiratory failure).
Type 1 respiratory failure is usually acute, and is caused by factors that impair oxygenation, although alveolar ventilation may be maintained. Examples would include low ambient oxygen partial pressure (e.g. altitude), and ventilation/perfusion mismatches such as consolidation (worsening shunt) and pulmonary embolism (worsening dead space).
Type 2 respiratory failure can be acute or chronic and reflects a failure of alveolar ventilation; consequently, paCO2 rises. Causes include central hypoventilation, chest wall abnormalities, neuromuscular disease and increased airways resistance.
Acutely raised paCO2 results in a respiratory acidosis with a reduced pH, due to the action of carbonic anhydrase catalyzing the following reversible reaction:

In chronic respiratory failure, there is renal compensation for the prolonged rise in CO2 via retention of bicarbonate (a base), to maintain a normal pH. This is seen as an increase in the bicarbonate level on the ABG with a positive base excess value.
Carboxyhaemoglobin (COHb) levels are often available on ABG reports and are a measure of the amount of carbon monoxide in the blood. COHb is not a sign of respiratory failure, but may be raised if the patient is a smoker. The normal value for non-smokers is 1.5–3%, but this can be increased to 5–15% in heavy smokers. Levels of 15–20% suggest significant carbon monoxide poisoning, as may occur after smoke inhalation. Levels over 40% indicate severe poisoning, with seizures and loss of consciousness likely.
Question 41: TFTTT
Fick’s law states that the rate of transfer of a gas through a sheet of tissue is proportional to tissue area (A) and the difference in gas partial pressures either side of the membrane (P1 – P2), and inversely proportional to the tissue thickness (T). The rate of transfer is also proportional to the diffusion constant (D), which is proportional to the solubility of the gas and inversely proportional to the square root of the molecular weight.

Where D∝SolubilityMW
Question 42: TFFTF
The A–a gradient is a measure of the difference between alveolar and arterial partial pressures of oxygen (pAO2 – paO2). It is used in diagnosing the cause of hypoxaemia; the measurement helps isolate the problem as either intra- or extrapulmonary. A normal A–a gradient for a young adult, non-smoker, breathing air is between 5 and 10 mmHg. It increases with age; a rough estimate can be calculated by (age in years/4) + 4.
An abnormally raised A–a gradient indicates that the hypoxaemia may be due to a defect in diffusion, V/Q mismatch or right-to-left shunt.
The A–a gradient is normal in hypoventilation and at high altitude, as the lung parenchyma can be assumed to be normal. Hypoxaemia in the face of a normal A–a gradient implies hypoventilation with displacement of alveolar oxygen by CO2 or another substance. At altitude, paO2 is low but only because pAO2 is low; transfer of gas is within normal limits.
Question 43: FTTFT
The oxyhaemoglobin dissociation curve is an important tool for understanding how our blood transports and releases oxygen. It specifically relates oxygen saturation and partial pressure of oxygen in the blood, and is determined by the affinity of haemoglobin for oxygen.
The affinity is affected by several factors; these shift or reshape the curve. A rightward shift indicates the Hb has a decreased affinity for oxygen, but it makes it easier for the Hb to release oxygen bound to it. The effect here is to increase the partial pressure of oxygen in the tissues where it is most needed. Conversely, a leftward shift indicates an increased affinity for oxygen so that Hb binds it more easily, but unloads it more reluctantly.
The term P50 is defined as the partial pressure of oxygen at which the oxygen binding protein (usually Hb) is 50% saturated. P50 is used to specify the position of the curve, i.e. it is a measure of oxygen affinity. The P50 is located on the steepest part of the curve and therefore shifting the curve left or right has a greater effect on SpO2 here. P50 is shifted to the right with an increase in hydrogen ion concentration, pCO2, temperature and 2,3-DPG.
In stored blood there is a decrease in 2,3-DPG levels, hence P50 is shifted to the left (increased affinity of Hb for O2).
In chronic anaemia there is an increase in 2,3-DPG, facilitating more tissue release of oxygen.
HbS has reduced affinity for O2, thus P50 is shifted to the right.
HbF has high O2 affinity and the curve is shifted to the left. There is also leftward shift in carbon monoxide (CO) ingestion as Hb binds with CO 200–250 times more readily than with oxygen.
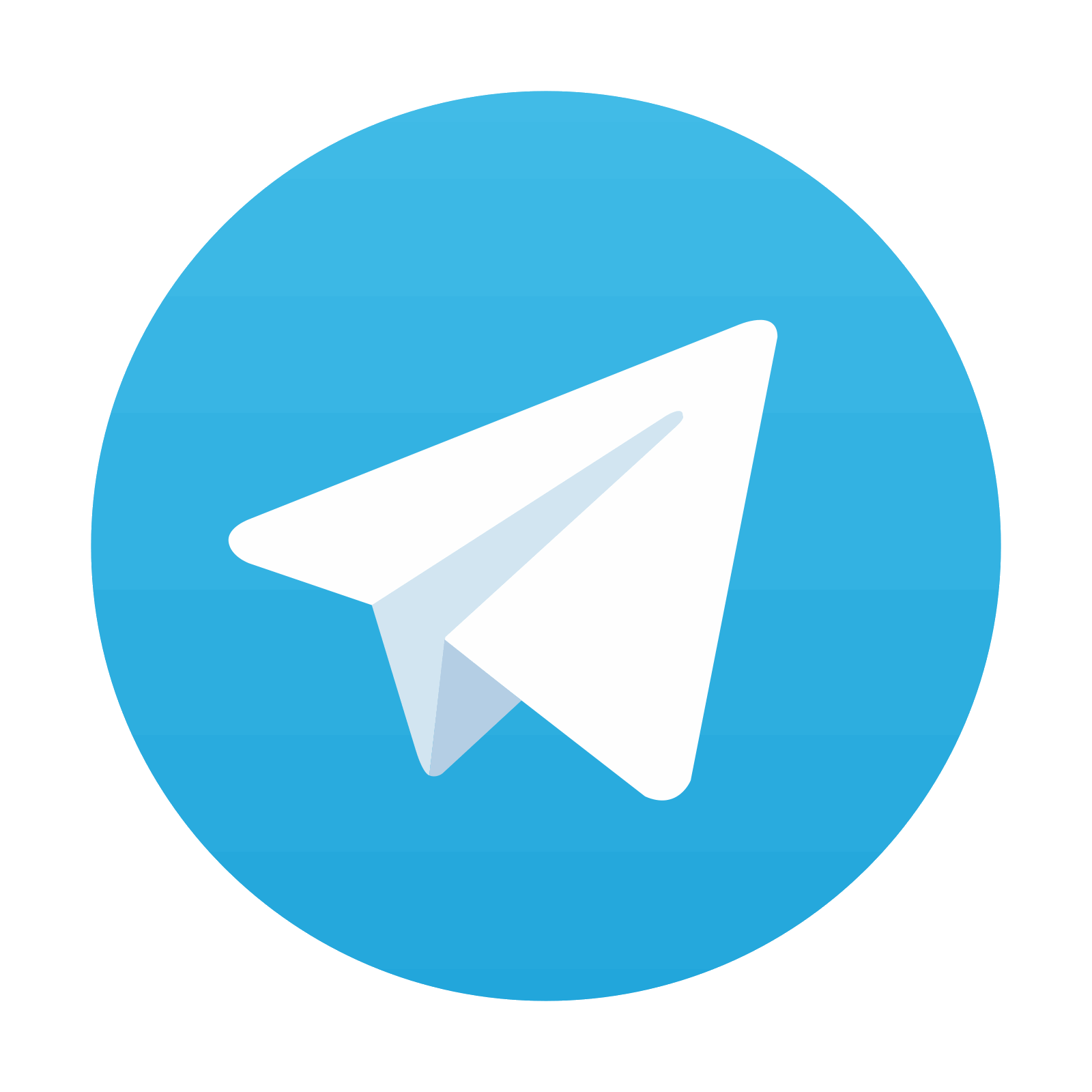
Stay updated, free articles. Join our Telegram channel
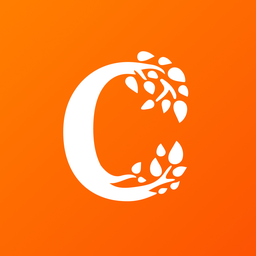
Full access? Get Clinical Tree
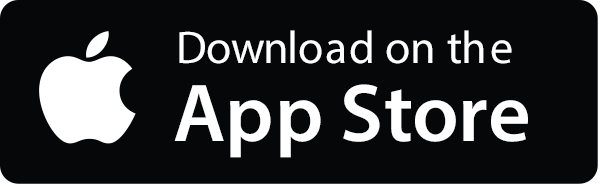
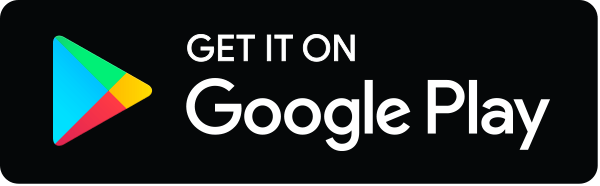
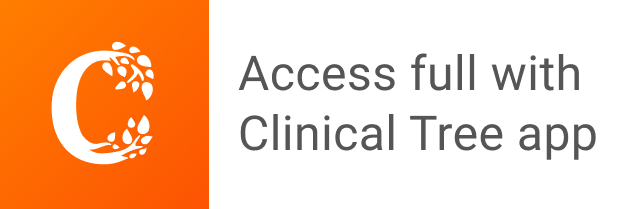